Theories of fusion and endosymbiosis
Back to main indexThis group of theories is not new. Indeed, the first formulation dates back to the beginning of the 20th century by the Russian biologist Konstantin Mereschkowski (1855-1921). There are some theories of this category that involve a single fusion or endosymbiosis event and others that involve more than one. They start from the observation that the mitochondria and the various plastids very probably derive from endosymbiosis events, and that mutualistic and parasitic intracellular endosymbioses still exist today. In addition, we have seen that the trace of a mixture of genes of various origins is detectable in the current genomes of eukaryotes. However, some researchers doubt the latter argument. They suggest that genes could have been acquired horizontally later in evolution, either via interspecific transfers or via food:
- Efficient systems of gene transfer between distant species (via conjugative plasmids) are frequent in bacteria. A few examples are known between bacteria and eukaryotes, for example between Agrobacterium tumefaciens and plants. Likewise, the role of viruses in gene transfer is poorly understood. For example, RNA polymerase in mitochondria is of the viral type, suggesting transfer from a virus: is this a unique event, or have such transfers occurred frequently during evolution?
- The genes could have been acquired through food. Since the eukaryotic cell has the ability to phagocytize prey and digests it in its cytoplasm, it is possible that the escape of DNA molecules from the digestion vacuoles to the nucleus could have allowed the introduction of bacterial or archaeal genes into the genome.
The extent of horizontal transfers during evolution is difficult to estimate. Recent evolutionary transfers have been detected in the majority of the genomes of present-day eukaryotes, suggesting that they may occur relatively frequently. However, such transfers do not seem to be able to easily account for the very large number of genes from archaea and eubacteria present in the current genome of eukaryotes. Moreover, these transfers do not explain why genes of eubacterial origin are essentially functional and those of archaean origin essentially informational. The current consensus, strongly supported by the fact that mitochondria seem to derive from α-proteobacteria, is therefore that the eukaryotic cell is an assembly of at least two prokaryotic cells: a eubacterium and an archaea. The current debate is centered on the fine details of this genesis. The theories are too numerous to be fully articulated, especially since some seem poorly supported by reasonable arguments. The model must take into account a number of constraints, including the need for a succession of plausible steps giving rise to natural selection easily and immediately and the fact that no biological membrane seems to be able to appear easily de novo. The main problem therefore comes down to explaining how the “nucleus/reticulum/golgi” system appeared. InFigure 19, three possibilities for the appearance of the nucleus are summarized. Of these three models, the invagination model is the most likely because it requires the fewest steps and perfectly captures the topology of the current eukaryotic cell. The other two models are more complex and require that certain organelle membranes result from the fusion of two membranes of different origins, which is less likely.
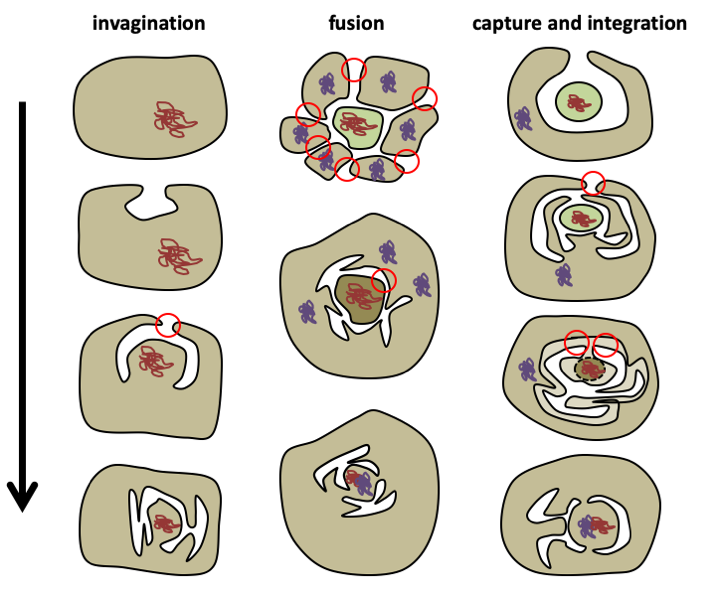
Figure 19.
Three models explaining how the nucleus could have appeared. The invagination model is the simplest because it assumes a single membrane fusion (represented by the red circle). The fusion model within a consortium of prokaryotic cells requires two stages of fusion events, creating the plasma membrane and then the nuclear pores. The capture and integration model requires two stages of fusion events and the disappearance of the membrane of the captured cell. In the invagination model, mixing of bacterial and archean genomes occurs at the time of mitochondria formation. In the other two models, the composite genome is formed before the arrival of the mitochondria.The invagination model also makes it possible to bring into play only a single major event to explain the entire “nucleus/reticulum/golgi” network, unlike the other models, for which the origin of the other organelles is less clear. Indeed, the nuclear double membrane would result from an endocytosis vesicle. The analysis of the molecular mechanisms regulating the pathways of exo- and endocytosis and the management of the nuclear membrane and the traffic through it shows that all involve small G proteins. There are 7 major families: Sar, Arf, SRb, Rab, Ran, Ras and Rho. They are ubiquitous in eukaryotes and are therefore ancestral. The continuity of the “nucleus/reticulum/golgi” system and the recurrent use of small related G proteins to manage the various organelles confirms a single origin for this whole network.
Although seemingly complex, the placement of endocytic vesicles is actually quite straightforward (Figure 20). The creation of an invagination of the plasma membrane requires only three partners: a small G protein which anchors to the membrane (this is possible because lipid groups are added to it post translation), a microtubule and a kinesin. This simple system reconstituted in vitro allows the creation of invagination of the plasma membrane. If the kinesin pulls hard enough, an internal vesicle can be created. The formation of such vesicles can be facilitated by a protein capable of constricting: actin. These can fuse with the plasma membrane again, giving rise to exocytosis vesicles. Regulation of vesicle formation was able to give rise to endocytosis and eventually the nuclear membrane was able to individualize around genetic material, which initially could be attached to the plasma membrane, as in bacteria. From the nucleus and/or exo-endocytosis vesicles, specializations have given rise to other organelles: reticulum, golgi, endosomes, peroxisomes etc. Each step could be accompanied by duplications of the genes of small G proteins, allowing confinement of each of them at precise levels of the network, and the appearance of proteins dedicated to the management and trafficking of the different organelles and vesicles: clathrins, syntaxins, ESCRTs complexes, etc. Genome analysis shows that all eukaryotes possess the basic machinery for organelle management, although later specializations through loss and gain of certain actors have occurred during evolution.
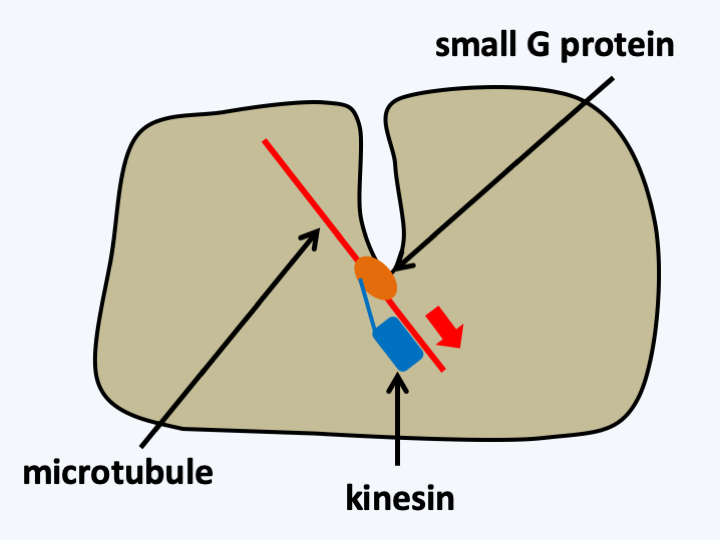
Figure 20.
Creation of plasma membrane invaginations. In vitro, the formation of an invagination requires only three components forming a complex: a small G protein, a microtubule and a kinesin. The kinesin attaches to the microtubule which provides it with a rail. The kinesin binds to the small G protein, which is attached to the plasma membrane via a lipid group added post-translationally. ATP provides energy to kinesin to exert the pulling force necessary to create the invagination. The formation of an internal vesicle only requires fusion at the level of the plasma membrane.Selection pressures favoring the formation of the “nucleus/reticulum/golgi” network are easily identifiable. The creation of invaginations leads to an increase in the exchange surface between the cell and its environment and the creation of a sheltered niche, which facilitates, for example, the possibility of exchanges with other microorganisms by giving them protection and ideal growth conditions. The establishment of the secretion system allows finer regulation of protein export and that of the endocytosis system allows non-soluble particles to enter the cell interior; it therefore allows a new mode of nutrition: phagocytosis. The cell would need to become larger in order to phagocyte its prey and therefore to have to partition the genetic material, thus creating a strong selection pressure for the establishment of a nucleus. This establishment is also accompanied by the improvement of the actin and tubulin cytoskeleton, which could then be recruited to participate in cell division, also allowing the increase in the size of cells and genetic material, and cell shifts using pseudopodia and flagella. In fact, in almost all major groups of eukaryotes there are amoeboid cells, which move by the actin cytoskeleton, or flagellates, which move by the tubulin cytoskeleton. In some groups, such as Myxogastrida or Heterolobosea, cells can switch between the two types of morphology depending on external conditions. This ability confirms that the two types of cytoskeleton are ancient, and suggests that the initial eukaryote could already have had both types of cytoskeleton.
The nature of the cell that underwent these transformations is unknown, but the discovery in Lokiarchaeota of actin, components of the ESCRT complex, etc. confirms the hypothesis of an origin close to the archaea. Phylogenetic analyzes based on the sequences of genomes from the three domains of life support this hypothesis and place the ancestor common to all eukaryotes or LECA (Last Eukaryote Common Ancestor) in the TACK super-phylum, near Lokiarchaeota (Figure 21). This cell endowed with movement and endocytosis, which we can now call a proto-eukaryote, is ready for the next stage of its evolution, which is the creation of mitochondria. This was probably done via the endocytosis of an α-proteobacterium capable of breathing. Two major groups of hypotheses consider two distinct types of selection pressures that allowed the establishment of the mitochondria.
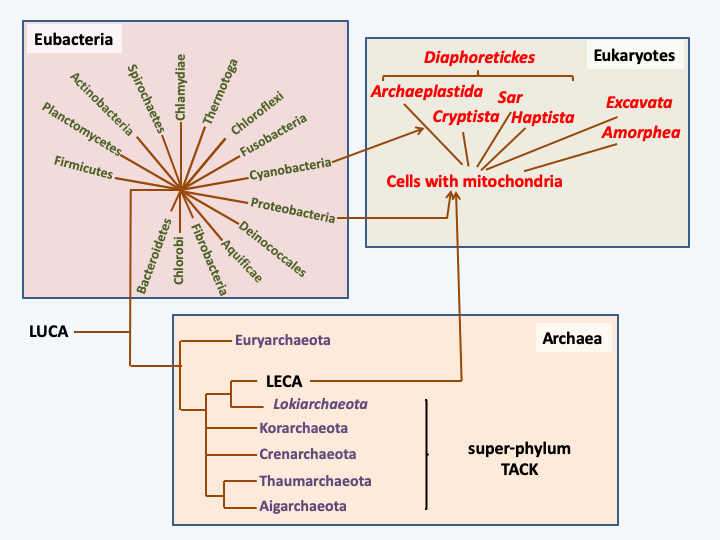
Figure 21.
Phylogenetic tree of living organisms constructed from genome sequences (phylogenomic tree). Eukaryotes are thought to be derived from the association of a cell related to the archaea of the TACK super-branch and an α-proteobacterium. The subsequent association of a eukaryotic cell with a cyanobacterium led to the emergence of a cell line with a so-called 'primary endosymbiosis' plastid.“Syntrophic” theories suggest a nutritional association between two partners, one of which feeds on the waste produced by the other. Several types of such mutualism involving different partners have been considered. In the example ofFigure 22, a hydrogen-producing bacteria is gradually incorporated into a cell that uses hydrogen as an energy source, like today’s methanogenic bacteria. The hydrogen-producing bacteria also had to be able to do respiration in order to account for the current role of the mitochondria. In these theories, the “nucleus/reticulum/golgi” network occurs concomitantly with or after cell integration. These theories do not provide obvious selection pressures to account for the genesis of mitochondria, nor for that of the cytoskeleton. An alternative theory postulates that the proto-eukaryotic cell would have been a predatory prokaryote of bacteria, which at the time was to be an under-exploited ecological niche. The mitochondria would derive from the abortion of a phagocytosis (Figure 23), hence the name “phagotrophic” given to this theory.
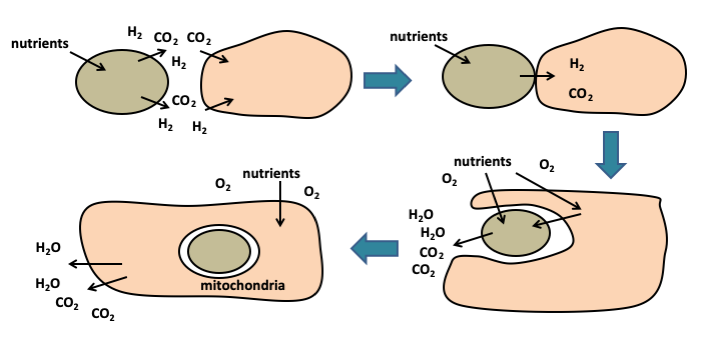
Figure 22.
Formation of mitochondria by syntrophy. The metabolic association of two cells, one of which consumes the other's waste, is called syntrophy. Optimization of syntrophy leads to an increasingly integrated functioning of the two cells, which has led to the internalization of one of them, to give the mitochondria. In this example, one partner produces hydrogen which the other uses as a source of energy. This type of association may have occurred in anaerobic environment, probably before the appearance of atmospheric oxygen from cyanobacterial oxygen photosynthesis. When it appeared, its toxicity led to the selection of cells capable of converting it into water, via respiration.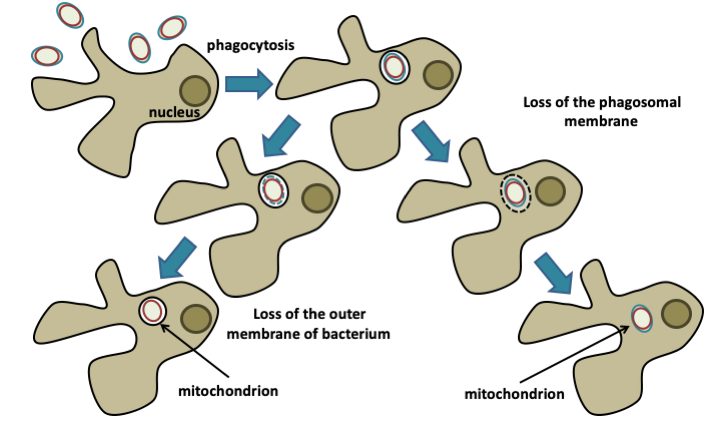
Figure 23.
Formation of mitochondria by phagotrophy. The protoeukaryotic cell is a predator that consumes the bacteria that surround it. The mitochondria would have appeared following an abortion of the digestion process, either by escape of the bacteria into the cytosol following the lysis of the phagocytosis vesicle (phagosome), or because of the resistance of its internal membrane to digestion, which would only have resulted in the disappearance of the outer membrane.The choice between the different theories is not straightforward, but I (the original author) would favor that of phagotrophic origin, as it relies on fewer steps, each of which may have been constrained by immediate and powerful selection pressure. It is perfectly in agreement with the current topology of the eukaryotic cell. It also helps explain characteristics of the eukaryotic cell, such as the central role of mitochondria in apoptosis or of sterols in membranes allowing their fluidity. Here is a plausible scenario for the emergence of the eukaryotic cell:
- At the beginning of the history of life, more than 2 billion years ago, the earth must have been populated by a large number of bacterial and Archean groups, only a part of which has survived to this day. These prokaryotes had to feed either by anoxygenic photosynthesis, such as that carried out by many eubacteria such as chloro- and rhodo-bacteria, by chemotrophy, such as bacteria and archaea present in hydrothermal vents, or organic molecules produced naturally by chemosynthesis or derived from cell activity. An ecological niche was therefore available: the use of other cells as a source of food. Different groups of bacteria have used and still use this resource. Bdellovibrio bacteriovorus, for example, penetrates inside other eubacteria, which it then lyses to feed on them. Myxobacteria, such as Myxococcus xanthus, behave like a “pack”, that is, they group together, move with the help of flagella to bacterial colonies and have the ability to secrete molecules that will lyse the bacteria they feed on. It is therefore possible that a group of archaea, related to the now defunct TACK superphylum, evolved this type of nutritional mode. This change of nutrition could have led this particular group of archaea to efficiently secrete proteins capable of killing other cells, favoring the establishment of the secretion system, but also to put in place efficient means to move and capture prey. These adaptations could have been made possible by a complexification of the cytoskeleton and the evolution of amoeboid and flagellar movement. This cell would also have undergone strong selection pressure to quickly and efficiently consume its prey, thus allowing the appearance of phagocytosis. Note that in order to be able to swallow prey, it is no longer necessary to have a rigid wall surrounding the cell. It is very likely that we will never know the exact appearance of these cells, but they could have looked like Gemmata obscuriglobus: cells without a rigid wall, with a fluid membrane thanks to sterols, moving through the sediments in search of prey that they kill, initially by secreting toxins, before gradually moving on to swallow the prey during evolution, in order to digest it more efficiently.
- In such a world, the prey does not remain defenseless, and it is quite possible that some had systems in place to subvert the phagocytosis, which they could have used as a gateway inside the predator. The proto-eukaryotic cell has itself become a source of food for its prey! Intracellular pathogens are therefore likely to have arisen very quickly during evolution. The world has then become complex: prey try to avoid predators who in turn try to avoid being parasitized! This is the eternal race of the Red Queen, one of the most powerful engines of evolution! On the one hand, the proto-eukaryote improves its movement system, in particular by developing a flagellum (Box 3), and control of prey bacteria when these are in its digesting vesicles; on the other hand, prey sets up efficient digestive escape systems by first lysing the digestion vesicle and secondly the proto-eukaryotic cell.
- An independent event occurred just over 2 billion years ago: the invention of oxygenic photosynthesis via the serialization of the photosynthetic complexes PSII and PSI by cyanobacteria. These cells become able to break water molecules, but at the same time release oxygen into the atmosphere. Oxygen is a violent poison. Today’s organisms have many systems that allow survival in the presence of molecular oxygen and its toxic derivatives: peroxides, superoxides, singlet oxygen, etc. It is very likely that two billion years ago many species did not have such systems and therefore disappeared, except in a few anaerobic biotopes. This ecological disaster is probably unparalleled. Even humans have yet to transform ecosystems so profoundly. The number of groups that have disappeared without a trace as a result of the onset of oxygen is probably very large.
- The gradual rise in the quantity of oxygen in the atmosphere therefore provides a strong and immediate selection pressure to stabilize a eubacterium capable of breathing oxygen - and therefore of converting O2 into water - inside the proto-eukaryotic cell. The eubacterium in question was an α-proteobacteria related to present-day rickettsiae, some of which are obligate intracellular pathogens. For example, Rickettsia prowazekii causes typhus. These parasites enter cells through phagocytosis which is followed by lysis of the phagosome (right diagram in Figure 23). It is therefore likely that the ancestor of mitochondria, or proto-mitochondria, was a cell already capable of surviving inside the proto-eukaryote and followed the same evolutionary path to remain in the cytoplasm.
- This protoeukaryotic + proto-mitochondria bicomponent cell was able to survive anaerobic and aerobic conditions. It was therefore able to colonize all environments and eliminate all competition: the eukaryotic cell was born. The proto-mitochondria are becoming more and more integrated; its mechanism for killing the host cell is recycled in programmed death processes: apoptosis. The eukaryotic cell continues its evolution by diversifying. Some continue to eat bacteria and reiterate the stabilization of the prey within the cell, but with cyanobacteria, giving rise to plastids. This line will give birth to the current plants that are so familiar to us. Others prey on larger prey: other eukaryotic cells. Another race of the Red Queen of which we are today one of the results was born. The following chapter details the different stages of this race in more detail.
Box 3. Eukaryotic flagellum
The eukaryotic flagellum is one of the most complex subcellular structures and its appearance raises several evolutionary questions. How could such a complex structure be set up? Particularly a structure that seems to provide a selective advantage - swimming in the water column - only when it is complete? How could the cell produce a flagellum by spontaneous assembly of molecules?
The answer to these questions comes once again from a progressive evolution of the flagellum, which in fact seems to have two functions: swimming, but also the detection of signals via membrane receptors. In the scenario proposed above, the proto-flagellum makes it possible to detect molecules that are secreted by potential prey, causing a deformation of the membrane subtended by microtubules and not microfilaments as in the case of pseudopodia. The first function of the flagellum would therefore have been sensory, but this extension would have quickly enabled, like the pseudopods, the cell to crawl on the substrate. Improvements in the structure result in the current flagellum which still retains these two functions. Consistent with this hypothesis, it would appear that some current eukaryotes use their flagellum to glide on the substrate and not to swim. However, this model does not capture the fact that early eukaryotes had not one but two flagella, nor that basal body duplication most often requires the presence of a preexisting basal body. This last observation has led to several biologists suggesting two additional hypotheses: the flagellum would come from an ectosymbiotic bacterium related to the Spirochetes or from a virus. There are currently eukaryotes that use such bacteria to move around, but the bacterial hypothesis is nevertheless not very credible. Indeed, how does the bacteria positioned outside the cell give rise to an intracellular organelle? The virus hypothesis is more credible but awaits confirmation. The virus would have provided the structure that initiates the polymerization of microtubules, in the form of nine triplets of microtubules and which holds the microtubules together.
Back to chapter index