The eukaryotic cell
Back to main indexEukaryotic cells (Figure 3) are often large in size and have a complex structure with a true cytoskeleton (microtubules of tubulins, actin microfilaments, etc.) and a system of internal membranes delimiting numerous organelles: nucleus, endoplasmic reticulum, Golgi apparatus, early endosomes, late endosomes or multivesicular bodies, lysosomes, vacuoles, peroxisomes, inclusion bodies, mitochondria… They are always surrounded by a single plasma membrane containing varying levels of sterols. Structures located under the membrane (the cortex) or outside (wall, thecae, frustules, shells, etc.) often strengthen the membrane and give it a well-defined shape. The cytoskeleton (Box 1) not only reinforces the cell to enable it to resist external attacks, but also allows the intracellular movements of various materials required by the large size and compartmentalisation of the eukaryotic cell, including vesicular trafficking (Figure 4), as well as the movements of the cell, either via the pseudopodia (Figure 5) based on the actin/myosin cytoskeleton or the flagella or cilia (Figure 6) based on the tubulin cytoskeleton. The topology of the organelle system, as well as the modalities of their formation and management, make it possible to differentiate at least two large subnetworks.
Box 1. The eukaryotic cytoskeleton
The cytoskeleton allows the eukaryotic cell to retain its shape but also, due to its dynamics, to change shape and move around. It is also involved during cell division to separate genetic material and enable intracellular trafficking. It is made up of 3 types of elements:
- Microfilaments produced by the polymerization of actin. A microfilament is about 8 nm in diameter. Actin is recruited from its monomeric form (G-actin) and is incorporated as F-actin. Actin is conserved in all eukaryotes and is used to (1) ensure the formation and transport of vesicles, (2) divide the cell into two daughter cells (the modalities of this role vary depending on the organism) and (3) generate pseudopodia for displacement.
- The microtubules resulting from the polymerization of α-tubulin/β-tubulin dimers in the form of protofilaments, associating in groups of 13 to form tubes with a diameter of 24 nm. Like actin, microtubules are conserved in all eukaryotes and participate (1) in the transport of intracellular cargo, (2) ensure the division of chromosomes during mitosis and meiosis and (3) participate in the development of flagella that allow swimming.
- Intermediate filaments which, unlike the previous ones, do not have the same composition in different groups of eukaryotes.
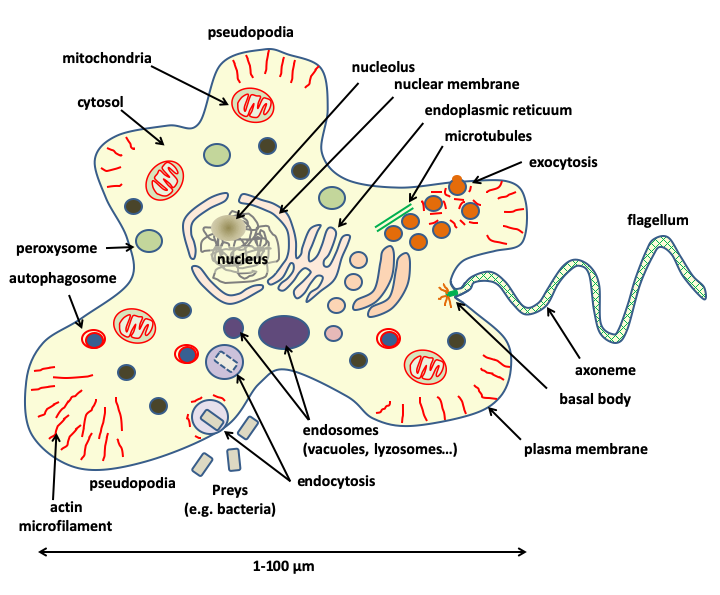
Figure 3.
Eukaryotic cells have a complex cellular structure associated with the presence of a true cytoskeleton and membrane-bound organelles, including a nucleus, which gives them their name. They are generally large in size: a eukaryotic cell is on average 10,000 times larger than a prokaryotic cell.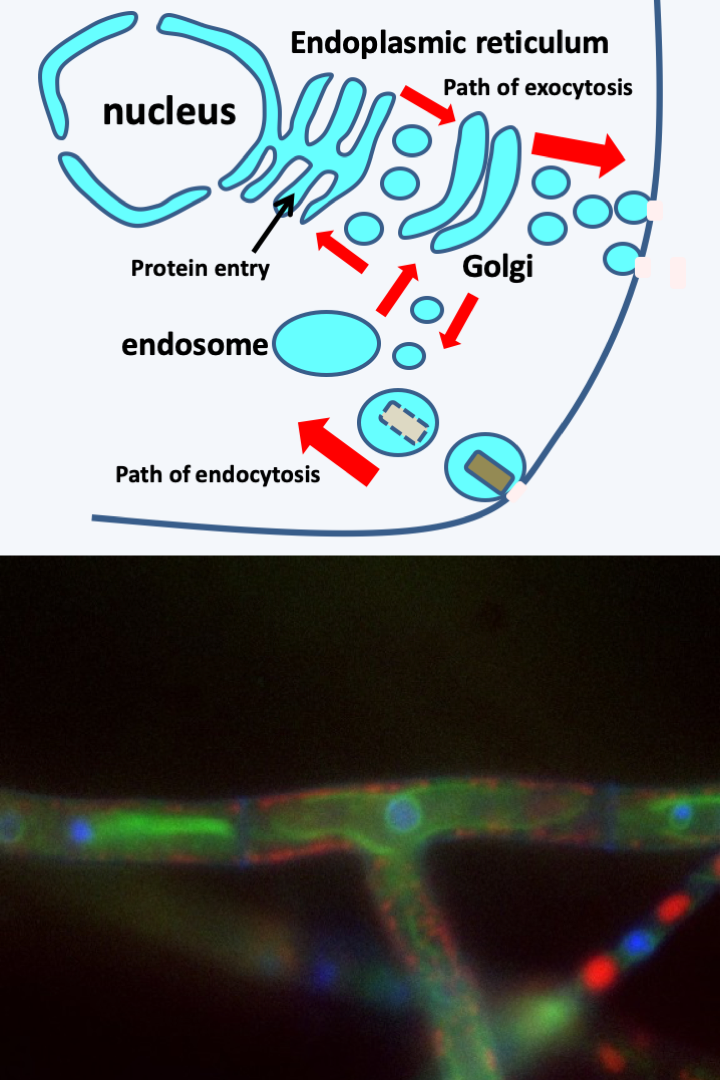
Figure 4.
Vesicular trafficking enables the movement of proteins within the cell by packaging them inside membrane-bound vesicles which can be viewed topologically as a continuation of the plasma membrane. The lumen of the vesicle would then be equivalent to the outside of the cell. In this way, proteins can be exported outside the cell via the endoplasmic reticulum, in green on the bottom image surrounding the nucleus in blue, then the Golgi apparatus. This pathway is called exocytosis. Vesicular transport also allows extracellular particles to enter the cell via vesicles, where they can be digested. This is the endocytosis pathway which ends in the endosome compartments: lysozomes, digestive vacuoles ..., in red on the bottom image. The red arrows indicate some of the routes that the vesicles can follow before merging with the different compartments. There are two particular types of endocytosis: phagocytosis where solid prey is ingested and pinocytosis where only liquid is incorporated. Other endocytoses do not allow the entry of extracellular material but allow, for example, the recycling of membrane receptors. Photo of a Podospora anserina hyphae: Sylvain Brun.First, the nuclear envelope and the endoplasmic reticulum are in physical continuity. Vesicles transport components internal to the reticulum to the Golgi apparatus and then to the exocytosis pathway (Figure 4). Conversely, the endocytic vesicles converge towards the endosomal compartment. This is in relation with the Golgi apparatus, and the other organelles, always via diverse vesicles (Figure 4). Likewise, several organelles surrounded by a single membrane, such as peroxisomes or inclusion bodies, derive by modification from vesicles arising either directly from the reticulum or from other components such as endosomes or Golgi. Some of these organelles, such as peroxisomes, have specific functions of sequestering dangerous metabolites, and others, such as lipid inclusion bodies, of storing nutrients. This first network also contains the autophagosomes although these are delimited by two membranes. Indeed, they are formed by elongation of vacuoles around the cellular components to be degraded. This “nucleus/reticulum/golgi” network should be compared with the sterol content in eukaryotic membranes. Sterols confer special biophysical properties, in particular, they facilitate membrane fusions and modulate fluidity and stability. Its management is under the control of small proteins hydrolyzing GTP (G proteins of the Rab, Ran, Rho family, etc.). The formation and transport of vesicles is mainly dependent on actin, although microtubules may be involved in transport. It therefore seems that this network can derive by successive specializations from the same initial organelle.
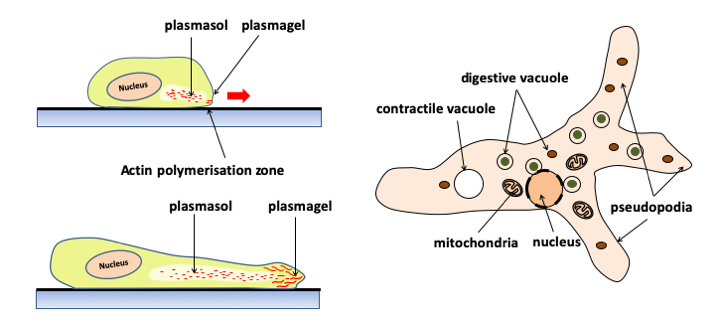
Figure 5.
Pseudopodia are dynamic extensions of the cytoplasm that allow cells to crawl around the substrate. Cells moving with pseudopodia are called amoebae and are said to be capable of amoeboid movements. Formerly, free amoeba was classified in the rhizopod class. This group is no longer considered valid because it is polyphyletic. Human leukocytes move by amoeboid movements. Pseudopodia are driven by waves of polymerization/depolymerization of actin microfilaments at the periphery of the cell; which consumes ATP. This creates directed cytoplasmic movements of the plasmasol (a state of the fluid cytoplasm without microfilament) located in the center, towards the plasmagel (more rigid because it contains polymerized actin) thus deforming the plasma membrane. The extensions of the pseudopodia due to the polymerization of actin, followed by their retractions caused by its depolymerization, ensure the movement of the cell. Pseudopods are often lobed (lobopods). In some cases, they are subtended by microtubules and can take particular very stretched shapes (filopods, reticulopods, etc.). These different forms are more or less specific for different groups of eukaryotes. The shape and number of the pseudopods make it possible to recognize the different species of amoeba. On the right a typical free amoeba, Amoeba proteus living in organic detritus at the bottom of ponds.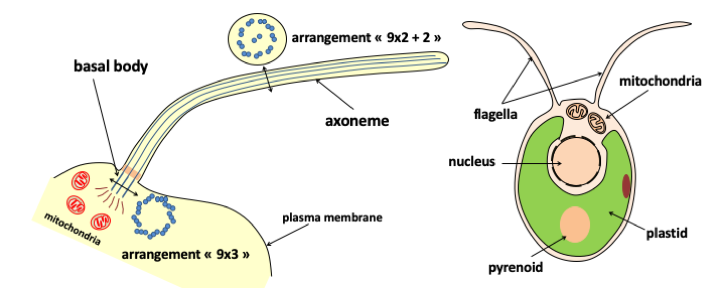
Figure 6.
Flagella are extensions of the plasma membrane composed of a specialized arrangement of microtubules. The beating of flagella allows cells to swim in the water column and therefore complement pseudopodia for locomotion. When theere are numerous, short flagella on the cell surface, they are called cilia instead. Protists that use flagella to move were previously classified in the flagellate group and those with cilia in the ciliate group. Howvere, neither of these two groups are monophyletic, and they have therefore been abandoned in the current phylogenetic classification. The flagellum is composed of two major parts: the axoneme and the basal body. The axoneme has an arrangement of nine peripheral microtubule doublets and a central doublet (9x2 + 2). These doublets are connected to eachother by nexin bridges and are associated with dynein. The hydrolysis of ATP by dynein causes a change in the conformation of this protein, creating tensions between the microtubules constrained by the nexin. The release of these tensions causes the flagellum to beat. The basal body anchors the axoneme to the cell. In the basal body, microtubules are arranged in nine triplets (9 x 3). This structure is identical to that of the centrioles. In fact, the basal bodies of flagella become centrioles during cell division. Centrioles have long been thought to be used to organize aster, the network of microtubules that is used to separate chromosomes. It would seem that in fact the centrioles do not serve to organize the microtubules but are associated with the organizing center of the microtubules to ensure their duplication and correct distribution among the daughter cells. Their main role therefore seems to be to serve as the basal body of the flagella. Between the two main parts of the flagellum, there is a transition zone whose structure is variable. The basal body is itself connected via fibers (the attachment complex) to other cellular structures. The shapes and distributions of these attachment structures differ among groups of eukaryotes. Intermediate pieces and attachment complexes serve as phylogenetic markers and support data from molecular phylogenies. On the right, the green alga Clamydomonas reinhardtii moves with the help of two flagella positioned at the front of the cell.Secondly, to this first network, we must add another one, formed by mitochondria whose genesis involves division from pre-existing mitochondria and which is not in connection via vesicles with the nucleus/reticulum/golgi network. The mitochondria has its own genome which participates, along with the nuclear genome, in mitochondrial biogenesis, which therefore takes place completely independent of vesicular transport. The mitochondria therefore form a second sub-network independent of that of the nucleus/reticulum/golgi. Finally, in algae and plants, there is an additional compartment with properties very similar to those of mitochondria: plastids, which like mitochondria have their own genome. These therefore form a third sub-network independent of the other two in photosynthetic eukaryotes, even if in some algae, it is embedded in the nucleus/reticulum/golgi network.
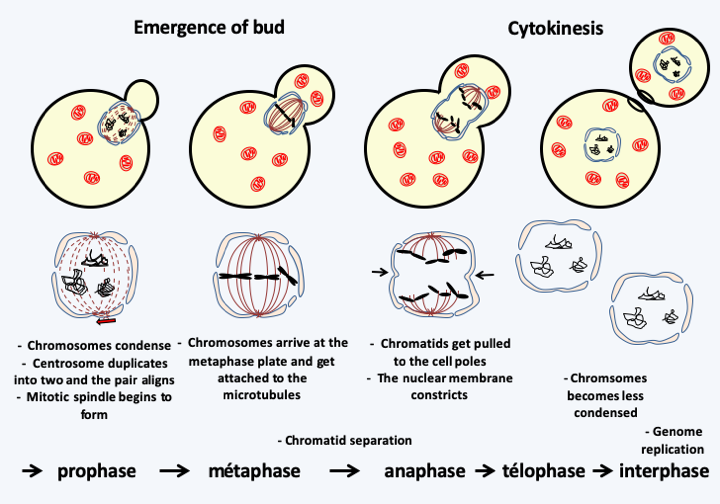
Figure 7.
The different stages of mitosis in a budding yeast show a concordance between nuclear and cellular divisions. This is not the case with all eukaryotes, where the two divisions can occur independently of each other. In many eukaryotic protists, such as budding yeasts, the nuclear membrane does not disappear upon cell division.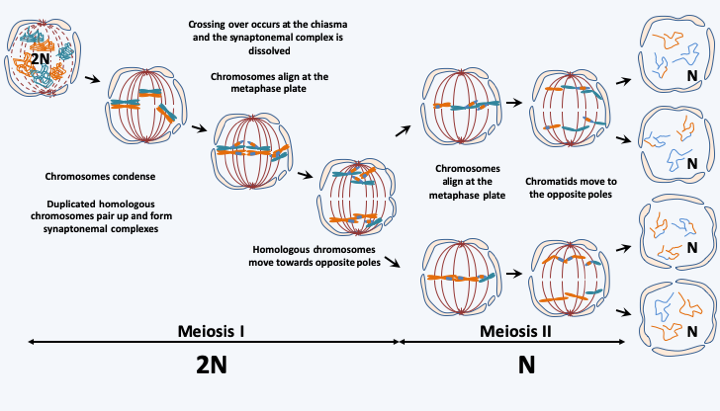
Figure 8.
Meiosis consists of two successive divisions resulting in four haploid cells from a diploid cell. The first division results in the separation of homologous chromosomes. It is at this stage that the two daughter cells basically revert to haploid, despite having their genetic material duplicated. They then undergo classic mitosis without genome replication to give 4 haploid daughter cells. The presence of genetic recombinations (crossing-over) and the random play of the distribution of chromosomes (shuffling) ensure that each of the cells thus produced is the unique result of a mixture of genes from the two parents who created the initial diploid cell.The presence of these organelles implies other functional consequences compared to prokaryotes. First, the presence of the nucleus allows additional steps in the regulation of gene expression. Indeed, the decoupling between transcription and translation as well as the splicing of the numerous introns allow the storage of the mRNA for later use, and to modulate not only the quantity of the mRNAs, but also their very nature thanks to alternative splicing. The nucleus contains most of the genetic material. It is probable that the sequestration of the genome in the nucleus made it possible to increase the cell size and therefore to make the eukaryotic cell even more complex, which in turn necessitated an increase in the complexity of the genome! Indeed, in eukaryotes, the size of the genomes varies from 2.2 Mb, a size smaller than certain bacterial genomes both in nucleotides and in number of genes, to the extraordinary one of 140,000 Mb, or 140,000,000,000 base pairs! Remember that the human genome contains about 3,000 Mb and codes for at least 25,000 genes and a large number of regulatory regions. Eukaryotic genomes are therefore often non-compact, with the presence of numerous introns, the combined size of which can sometimes exceed that of exons, and of selfish repeats such as transposons. Unlike prokaryotes, genes are not grouped into operons and polycistronic mRNAs are rare. However, in some groups of eukaryotes, genes can be co-transcribed and the pre-mRNA thus obtained is fragmented before translation. Finally, nuclear genetic material is present in the form of several linear chromosomes which adopt a very complex chromatin and supra-chromatin structure, in particular at the time of cell divisions. The processes of DNA partitioning are indeed much more complex than in prokaryotes. In general, division occurs by mitosis (Figure 7), and at the time of sexual reproduction in the form of meiosis (Figure 8). However, there are exceptions, for example in Ciliophora and Dinoflagellata which present amitotic-type partitions of nuclear material. The origin of mitosis and meiosis is still obscure. Indeed, if the genes involved in DNA replication and recombination (crossing-over) are present in prokaryotes, how did those involved in chromosome compaction, chromatid cohesion, microtubular network, cell fusion appear? Likewise, we do not clearly know the selection pressures that set up sexuality, because it does not have an immediate advantage, nor why some eukaryotes have lost it! Sexuality has three specific consequences, among other things. First, eukaryotes exhibit an alternation of haploid and diploid generations (Figure 9). As the genomes are different between haplophase and diplophase (see Box 2), alternation often makes it possible to express very different morphological and/or biological characteristics between haplophases and diplophases, ensuring a significant complexity of life cycles. In particular, the possibility of vegetatively growing via mitoses in each of the phases may vary depending on the species. Species with a majority haplophase are said to be haplobiontic (or haplophasic), those with a majority diplophase are called diplobiontic (or diplophasic), and those where the two phases have approximately equal durations haplo-diplobiontic (or haplo-diplophasic). Note that in Figure 9, plasmogamy and karyogamy, which are the two stages of fertilization, are dissociated. Indeed, there are organisms, like the Dikarya fungi, in which these steps can be separated by cell divisions that can occur for a very long time. During this dikaryotic phase, different characteristics of haplophase and diplophase may be expressed.
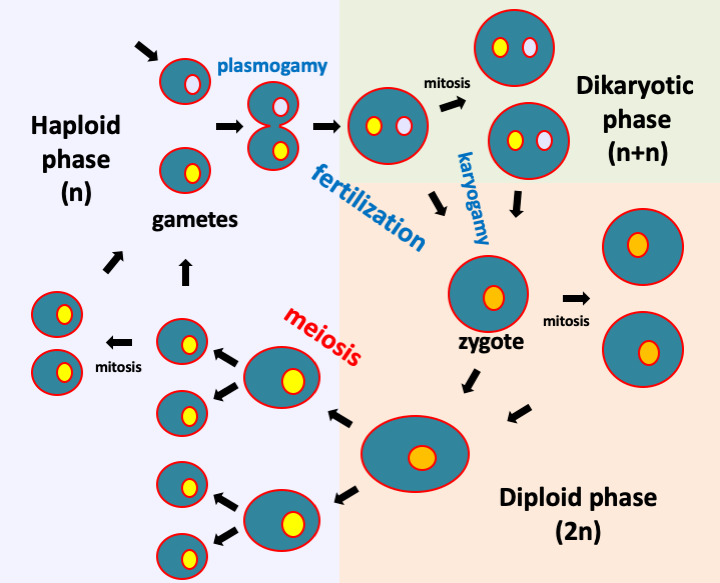
Figure 9.
The typical life cycle of a eukaryotic cell has two major phases; a diploid phase in which the cells are diploid and a haploid phase with haploid cells. Meiosis connects the diploid phase to the haploid phase of the life cycle. Fertilization (or conjugation) returns the cell to diploidy. This stage of the cycle first requires the fusion of the two cells or plasmogamy during which the cytoplasm are mixed, and then karyogamy which sees the fusion of the two nuclei. In some organisms, plasmogamy and karyogamy are decoupled and an additional stage of the cycle takes place: dikaryotic phase.Plasmogamy can occur between identical or different gametes (Box 2). There are two types of incompatibilities between mutually non-exclusive gametes:
- Morphological incompatibility is often manifested by the need to merge a large, most often immobile, called female gamete, and a small, generally motile gamete, called male gamete. These gametes can be produced by two different individuals (called sexual) or by the same individual (called hermaphrodite). Rather, conjugation is called plasmogamy of two morphologically identical gametes, while fertilization is a more general term.
- Genetic incompatibility manifests itself in the impossibility for two genetically identical gametes to fuse; these can have different or identical morphologies. This situation can be found in different strains within the same species. Strains for which the gametes have no genetic incompatibility and which are therefore self-fertile are called homothallic, and those which, on the contrary, exhibit genetic incompatibility, thus being self-sterile, are referred to as heterothallic. In the latter case, mating types are defined. Individuals can only reproduce between compatible mating types. Most often there are two. This is called bipolarity and crosses are therefore possible between individuals of different mating types and impossible between individuals of the same type. Genetically, the mating type is dependent on a single locus with two idiomorphs. We speak rather of locus and idiomorph because often the genetic determinism is more complex than a gene with two alleles. In some species, the mating type is determined by two idiomorphs (tetrapolarity) having several alleles. Hundreds of combinations can be defined and the compatibilities between them are often complex with several thousand mating types! Sexual incompatibilities therefore increase the complexity of eukaryotic life cycles by introducing partners that are potentially different in terms of their morphology, their genetic characteristics or at both levels!
A second difference with prokaryotic cells, which allows the internal network of organelles to exist, is the possibility of compartmentalization of energy production by mitochondria and plastids and of enzymatic reactions between cytosol, peroxisomes, lysosome etc., as well as of storing energy reserves more effectively than in prokaryotes. Eukaryotic cells are therefore not optimized for immediate energy use, which probably allowed their complexification and participated in the emergence of multicellular organisms.
A final important characteristic of eukaryotic cells intimately linked to vesicular transport is that they have the possibility of endocytosis (Figure 10) and therefore of ingesting solid particles of large sizes. It is a fundamental property of eukaryotic cells that distinguishes them from prokaryotic cells. We will see that it is probably at the origin of their genesis and plays a very large part in their evolution because it allowed and still allows the entry of living organisms into the cell. If they are not digested, these can then behave like parasites, but can also establish more harmonious relations, persisting in the cell and giving it a selective advantage. We will see that sometimes, such endocytosis results can become integral parts of eukaryotic cells during long-term evolution.
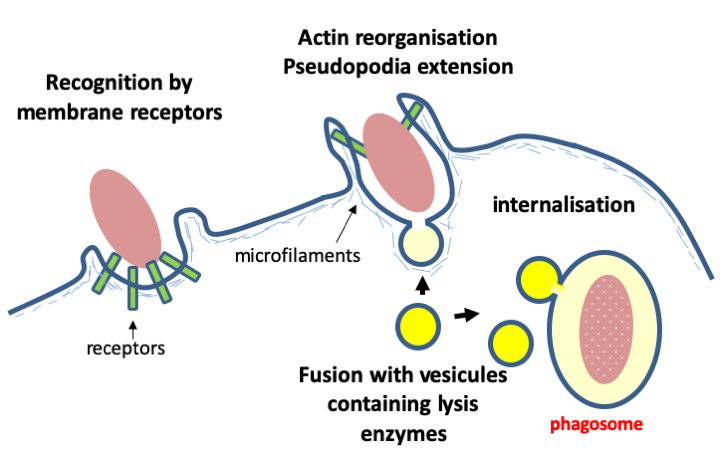
Figure 10.
The ability to ingest solid particles is a characteristic unique to eukaryotes arising from possession of the nucleus/endoplasmic reticulum/golgi network. It seems that this is an ancestral property that has allowed them to feed on prey that they recognize via specific membrane receptors. The mechanism of internalization is based on rearrangements of the actin cytoskeleton similar to what occurs at the time of pseudopodia extension. Prey is digested by fusing the phagosome with granules containing hydrolases. In some eukaryotes, phagocytosis, but not endocytosis, has been lost, allowing the development of a wall surrounding the cell. Thhas occured, for example, in plants and fungi whose trophic strategies (photoautotrophy and osmotrophy) make it unnecessary to ingest prey, making phagocytosis superfluous.Box 2. Ploidy levels
During their life cycle, eukaryotes have different genomes, which can have consequences on their phenotype. First, ploidy affects genome expression, and haploid and dikaryotic cells may not produce mRNA in the same proportion as diploid cells. One of the consequences is that diploid cells often are larger than haploid cells.
Second, diploid cells are the result of the fusion of two cells that can carry genetic differences. For example, in humans, haploid cells have either an X chromosome or a Y chromosome, while diploid cells are XX or XY. This last category, called heterozygous, clearly cannot be found in haploid cells! This case is general in heterothallic organisms which generally carry specific genes defining gender identity or species which have sex chromosomes that are responsible for morphological incompatibility during fertilization. The diploid is then heterozygous for the mating types or sex chromosomes while the haploids are homozygous.
Finally, in some eukaryotes, the nucleus can undergo changes in content or structure during the life cycle. Indeed, loss or amplification of DNA is observed in certain cells of multicellular organisms. Such cells are no longer intended to be used for reproduction (somatic cells) while germline cells intended for sexual reproduction retain all the genetic material. The most extraordinary case is that of the Ciliophora (Box 21), where expression is suppressed in one nucleus until the time of meiosis (called the germinal micro-nucleus) while cellular functioning is controlled by a giant nucleus (the macro- or somatic nucleus). The macro-nucleus derives from a micro-nucleus by amplification of certain regions of the genome and loss of other regions! Unlike the micronucleus, the macronucleus does not divide by classical mitosis but by nuclear fission. This case is not unique. It has been shown that in the yeast Schizosaccharomyces pombe, the microtubule spindle is not essential for division, in its absence the nucleus of this yeast divides according to a process of nuclear fission which could be the vestige of nuclear division before the invention of the microtubule spindle. Other types of non-canonical nuclear divisions exist, for example, in organisms lacking histone and whose genome does not compact in a classical way, such as Dinoflagellata (Box 23).
Back to chapter index