Eumycota
Back to main indexJump to section:
Introduction
The Eumycota, which means “real fungi”, constitute one of the eukaryotic lineages which has seen the greatest evolutionary success. It is also one of the best known to the general public (Figure 108) because it contains all the large fungi or “macromycetes” (= “large fungi”) that we consume, the yeasts that we frequently use in cooking to make bread, or that we have domesticated to produce alcoholic beverages such as wine and beer, but also most of the molds or “micromycetes” (= “small fungi”) that ferment or rot our food. It is also in this group that the fungi responsible for fungal infections and certain mildews that affect our crops are classified. The exact number of Eumycota species is currently unknown. About 100,000 species have been described, but it is likely that there are at least 1 or 1.5 million; maybe even 10 million (see here for the reasons of such disparate estimates). A typical forest or meadow soil can contain up to several hundred species. With current molecular techniques, it is possible to routinely identify around one hundred Eumycota species from ten grams of soil, thus making up the majority of species in the sample. This finding suggests that several hundred species coexist in one gram of soil, in the form of hyphae but also of spores. Collectively, they form a considerable biomass that is estimated to be much greater than that of metazoa! In particular, fungi often represent more than half of the total biomass (excluding root biomass) at the level of the upper soil layer, i.e. the first meter of depth. Bacteria represent only ten to twenty percent and animals about ten percent; the remainder being various other eukaryotic protists. In healthy forest soils and in decaying freshwater matter, the two biotopes in which fungi are the most common, they can represent up to 90% of the biomass. They occupy all biotopes, in high mountains on rocks and under snow, on the ocean floor where a great diversity of Eumycota fungi has been found, in ecosystems associated with ocean ridges, in coral reefs, in fresh and salty waters, including the Dead Sea, on the joints of your dishwasher… While the temperature optimum for fungi is generally around 20°C, some species are capable of growing at temperatures below 0°C and others of living at high temperatures, being the most thermophilic of eukaryotes. For example, the optimum growth rate for species living in compost is at around 55°C. Some spores of species that colonize fire-ravaged environments can survive exposure well above 100°C. Fungi often require a pronounced humidity (especially for reproduction), however some species can grow in environments with low “water tension” (0.62 for Aspergillus echinulatus while most plants die from 0.98). In terms of pH, some species tolerate very acidic environments (pH <3) and others very basic environments (pH >9).
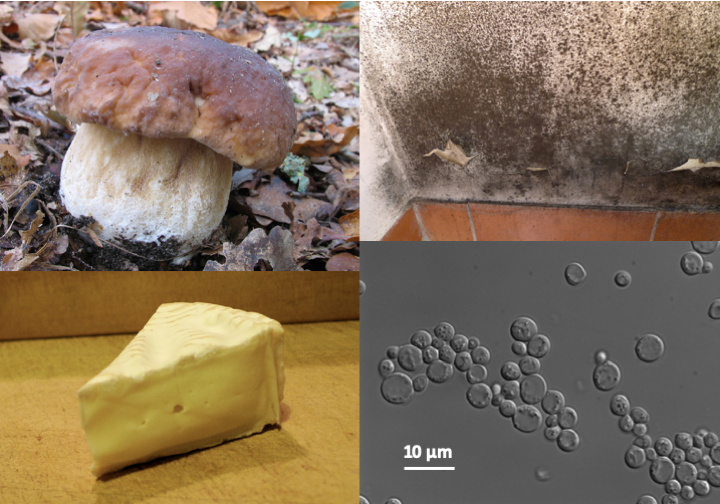
Figure 108.
Some known Eumycota. Top left, the Bordeaux cep, Boletus edulis; bottom left, Penicillium camemberti covering camembert; top right, a wall attacked by a mold, probably Stachybotrys chartarum; bottom right, the yeast Saccharomyces cerevisiae used to cook leavened doughs and make alcohols by fermentation.The nutritional strategies of Eumycota (Box 13) are varied but they are all based, with one exception (Geosiphon pyriforme, see Box 10), on an osmotrophic type diet. This is the major characteristic that directs the biology and evolution of Eumycota. Indeed, freedom from phagotrophy allowed them to protect themselves by a wall, which in Eumycota is composed mainly of chitin associated with α- and β-glucans. For this, they use specific chitin synthetases, some of which are type IV. It is difficult to trace the reason for the loss of phagotrophy. Two theories clash. The first suggests that the ancestors of the Eumycota were parasites, whose lifestyle often results in the loss of phagotrophy. Phylogenies support this theory, as the closest relatives of Eumycota are all parasites. In addition, type IV enzymes are also found in Rozella allomycis and Microsporidia. The other theory states that the fungi were originally saprotrophs which later became parasites. In fact, the majority of species of fungi, including many species considered to be the most primitive, are saprotrophs. However, rapid change in trophic strategy is a hallmark of the evolution of Eumycota (Box 13). The stabilizing cell wall gives cells their shape and allows them to effectively invade liquid and solid media in addition to offering protection. For example, there is no known fungal virus having an extracellular phase. The main evolutionary trends are therefore the increased capacity to degrade organic matter which is indigestible for other living beings, in particular lignocellulose, and efficient modes of dispersion, in particular in the air, because like animals and plants, Eumycota have emerged from the water to invade terrestrial ecosystems.
Box 13. Nutritional strategies employed by fungi
The Eumycota have adopted a wide variety of lifestyles during their evolution. Saprotrophs (synonyms: saprobes or saprophytes) feed on dead organic matter. They occupy an important ecological niche, that of recyclers of organic materials, most often difficult to degrade substances of plant origin such as cellulose or lignin. The majority of this activity takes place in temperate soils, which contain the greatest biomass and diversity of these fungi. They are the basis of various secondary food chains, beginning with the dead plant matter that is recycled by these fungi, which are themselves in turn eaten by insects, slime molds, etc. The emergence of the fungi capable of effectively breaking down lignin (in particular, Basidiomycota with the ability to cause brown and white rot) 300 million years ago coincided with the end of heavy deposits of carbon, suggesting a causal relationship between the two.
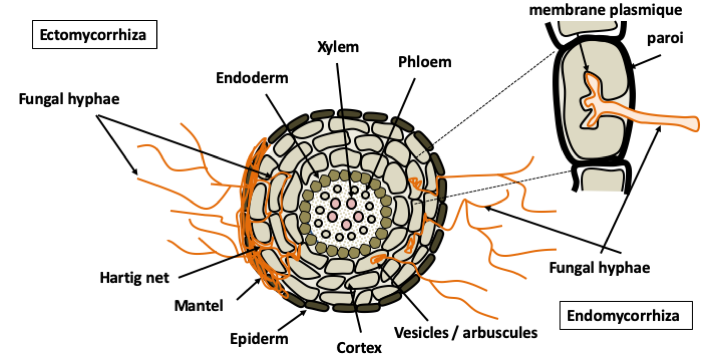
Many Eumycota live in association with photoautotrophs which directly provide them with carbonaceous and/or nitrogenous nutrition. Some live in symbiotic association with algae to form lichens for which several tens of thousands of species are known. As these organisms have been studied extensively by botanists, it is considered that almost all morphospecies are currently described, which is not the case for other trophic groups. Plant pathogens have developed parasitic lifestyles. The vast majority of diseases of cultivated and wild plants are caused by Eumycota fungi. Other fungi have associations in the forms of commensals or mutualists. Endophytes live entirely inside plants. On the contrary, mycorrhizal species develop a mycelium which is partly in the soil and partly in the plant. They form special structures with the roots, mycorrhizae. There are several types of mycorrhizae; the two most important are ecto- and endomycorrhizae. In all cases, the fungus lives in the root cortex without passing through the endoderm. The plant provides products of photosynthesis and the fungus provides mineral salts, nitrogen, and phosphate. It also protects against desiccation and pathogens that penetrate through the root. In ectomycorrhizae, the fungus surrounds the root in a mantle and inserts itself between the cells of the cortex to form the Hartig network, which is the place of exchange between the plant and the fungus. This type of mycorrhiza appeared late in evolution, but as it occurs with most trees in boreal forests, it has a very important role in ecosystems. In endomycorrhizae, the fungus penetrates the root and passes through some cell walls, but without entering the cytoplasm of the root cells. The exchanges take place at the interface of the membrane of the plant cell with the fungal hyphae. This type of mycorrhiza is present in almost all terrestrial plants and is made with specific groups of Eumycota: Glomeromycota and Endogonales. Plants without mycorrhizae have evolved recently and their ancestors had mycorrhizae, indicating that this association is very old.
A few genera of Eumycota have specialized as parasites or mutualists of animals such as the fungi of the digestive tract of ruminants or those cultivated by ants and termites. Note that as the temperature optimum for the vast majority of fungi is around 20-25°C, they are not very efficient (with a few exceptions) to invade and maintain themselves in warm-blooded organisms. In fact, the illnesses they cause, called yeast infections, often affect people with compromised immune systems. The most surprising way of life is that of fungi which, like carnivorous plants, supplement their nitrogen diet by capturing and consuming small animals and protozoa, especially nematodes, which they capture by means of various traps: sticky hyphae, lasso hyphae... Once captured, the prey is invaded by hyphae, killed and consumed by osmotrophy. This predatory behavior has been invented several times during evolution! Besides animals, fungi also parasitize other fungi. Certain lines, like that of Trichoderma, have specialized in this way of life.
Most fungi can use more than one of these nutritional strategies. For example, many plant pathogenic species can live in the bad season as saprotrophs on plant debris. Some saprotrophs live the beginning of their life as endophytes and invade the plant when it dies. Note that the reuse of the same nutritional strategies by fungi belonging to different phylogenetic groups is very common in Eumycota. The same is true for many morphological and/or physiological characteristics. These evolutionary convergences are obviously imposed by the similar life strategies of these organisms which, subjected to identical selection pressures, evolve towards the same types of adaptations. This diversity of nutritional strategies explains their evolutionary success and their significant biomass because they can use virtually any organic matter, living or dead, even if it is difficult to digest, even toxic!
Most fungal species have an intracellular structure typical of eukaryotes with a nucleus whose envelope generally does not disappear during division, an internal membrane network (reticulum, Golgi, endosome …), mitochondria and a classical cytoskeleton. However, in many species there is a decoupling between karyokinesis or division of the nucleus and cytokinesis or cell division. The cells are thus plurinucleated and therefore often adopt a so-called syncytial or coenocytic form. The cells are in most cases surrounded by a rigid and solid wall, the composition of which varies according to the groups of Eumycota. In most species, chitin and β-glucans serve as a backbone to which is added a variable matrix composed mainly of α-glucans and/or mannoproteins. The wall results from the deposition of materials produced in the cell and from the synthesis in situ once the skeleton is in place. The plasma membrane of Eumycota contains several sterols specific for each of the major lineages. The most famous and widespread is ergosterol which is one of the targets of antifungals and which is used to estimate fungal biomass in samples. Although essential, the role(s) of different sterols in hyphal biology is not yet fully understood.
A characteristic of fungi is their great morphological plasticity. First, their life cycle is divided into three stages which have very different morphological aspects (Figure 109). Fruiting bodies, also called sporophores or carpophores, and spores are involved in dispersal, while the thallus is responsible for the vegetative part of the life cycle. Many species appear to us mainly via their reproductive structures, such as the carpophores of macromycetes in autumn. Most fungi that humans harvest for consumption or other use are thus at fruiting stage, which is often very ephemeral. Being the most conspicuous fungal structure, the fruiting bodies and spores have for a long time governed the classification of fungi, especially since they are relatively constant for a given species. However, fungi are often able to produce very dissimilar sexual and asexual structures, which have led to two names, or even more, being given to the same fungus (Figure 76). Box 14 gives a small overview of the diversity of sporophores and spores, and the main categories defined by mycologists.
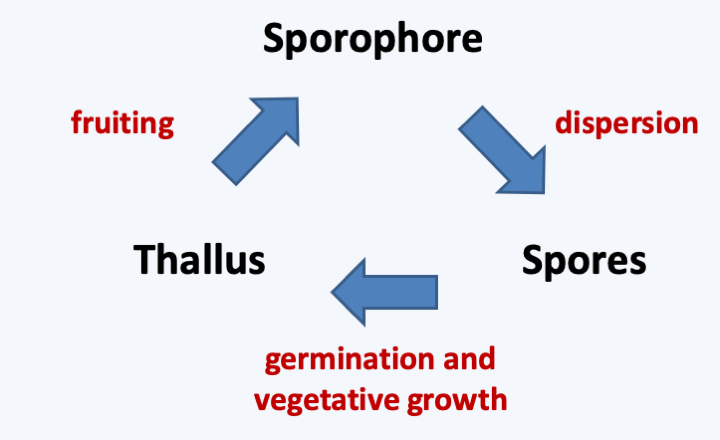
Figure 109.
Typical life cycle of a Eumycota.Box 14. Fungal sporophores
Fruiting bodies or sporophores have long served as a basis for the classification of Eumycota because their shapes and sizes are very varied, ranging from a few microns to several tens of centimeters. Fungi that produce large fruiting bodies, that is, one centimeter or more, are called 'macromycetes', the rest being called 'micromycetes' (keep in mind, these terms have no phylogenetic value but are purely descriptive). These fruiting bodies can be unicellular or multicellular, and in the latter case they are formed of more or less differentiated hyphae aggregating to each other. They can be the site of sexual or asexual reproduction. In the fungal phyla Basidiomycota and Ascomycota, the sexual fruiting bodies are called 'carpophores' and they are associated with complex terminology. For example, the sexual fruiting body of Basidiomycota (what is commonly known as thae mushroom) is referred to as a basidiocarp or basidioma, and that of Ascomycota ascocarp or ascoma. The tissue in which meiosis and spore differentiation occurs is called the hymenium. The shape of this hymenium makes it possible to define different types of carpophores. Agarics have a hymenium in the form of gills, boletes and polypores in tubes, etc.
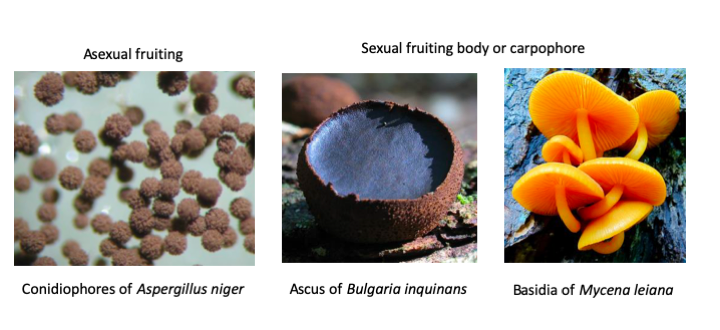
The evolution of carpophores has seen plenty of convergence, so that the old classification of fungi has been completely overhauled by the arrival of sequencing data since the 80s. Sometimes very surprising kinship relations have emerged. Nevertheless, molecular phylogenies have helped explain differences in the ontogeny of carpophores that have long mystified mycologists.
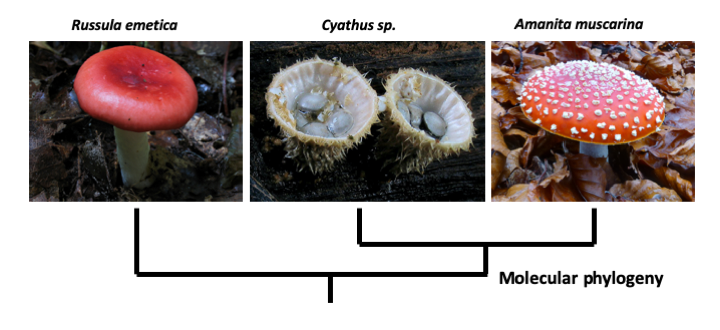
Eumycota spores exhibit a variety of origins and morphologies that have enabled their use in classification. Those resulting from meiosis are called meiospores and those resulting from mitosis are called mitospores. The mode of differentiation of the meiospores was the basic characteristic which served to differentiate each of the major lineages of Eumycota. Basidiospores are specific to 'basidiomycetes', ascospores to 'ascomycetes' and zygospores to 'zygomycetes'. This old classification agrees with molecular phylogenies reasonably well. Indeed, while the zygomycetes have for the moment disappeared from the classification, the Ascomycota and the Basidiomycota are still present and differentiated among other things by their meiospores. The spores are either provided with the posterior flagellum typical of Opisthokonta and are called zoospores, or are devoid of it and called aplanospores. The former are produced by fungi that have remained in the aquatic environment while the latter are formed by terrestrial fungi. Zoospores are metabolically active and primarily serve for dispersal. Aplanospores are involved in dispersal or persistence, and are metabolically inactive except at the time of germination. In either case, the spores are unable to absorb nutrients and must therefore live on their reserves. They can be uninucleated, or plurinucleated, or even multicellular. The production methods of these spores (differentiation at the end of a hypha for chlamydospores, on a particular structure for conidia, by differentiation of a cell in the middle of a hypha for arthrospores, etc.) and their extremely varied forms often make it possible to identify the species which produced them.
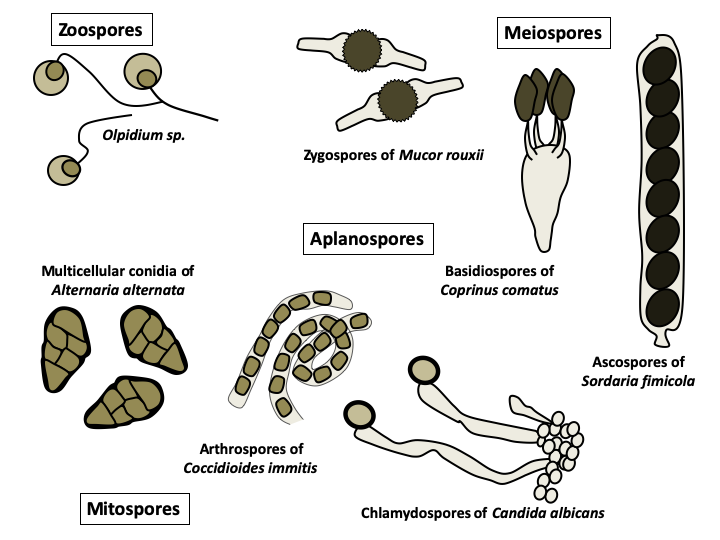
Spores can be directly obtained from the fruiting bodies. There are also devices that allow you to sample airborne spores. Spores can represent up to 45% of airborne particles. It is estimated that around 50 million tonnes of spores are released into the atmosphere each year, making it a major source of organic aerosols! The average frequency of airborne spores is 10-20 per liter of air but in some cases can exceed 1 million per liter, especially in the fall. They can then trigger allergic reactions or participate in the formation of clouds and rain. The germination of the spores restores the vegetative part of the fungal life cycle. This stage can be difficult to obtain, as most spores germinate only under specific conditions. The conidia (= asexual spores produced by budding off of hyphae) of Aspergilli germinate in a medium that contains glucose, and obtaining mycelium is therefore simple. The spores of phytopathogenic fungi usually germinate when they land on a hydrophobic surface, and those of most coprophilic fungi do not germinate in nature until after passing through the digestive tract of a herbivore. The failure rate for germination in the laboratory of these spores is therefore high, but some of these fungi are nevertheless cultivated quite easily. In contrast, the spores of Amanita have never been successfully germinated in the laboratory. Likewise, development of fruiting bodies can be very difficult to achieve. In fact, humans end up cultivating relatively few edible species. The vegetative form is usually easier to domesticate. For example, cells taken from fruiting bodies under sterile conditions often give rise to cultures which can then be propagated. Likewise, deposition of e.g. soil samples on axenic media results in the growth of fungi from the sample (Figure 69). Finally, placing specific “bait”, such as hair or other particular carbon sources, in the soil often allows the capture of mycelia for species that grow slowly but have a particular nutritional regime. Nevertheless, the direct cultivation of the vegetative form is sometimes very difficult, because certain species require very specific environments and are often in competition with more vigorous species. In addition, certain parasitic or mutualistic symbionts cannot grow without their partner and their culture has therefore never been obtained in vitro in an axenic medium. In summary, the majority of species could never be cultured from spore to spore in the laboratory. In contrast, some species can be grown very easily on simple media and have short life cycles, which makes them good study models: Saccharomyces cerevisiae, Neurospora crassa, Podospora anserina, Coprinopsis cinerea, Aspergillus nidulans, Sordaria macrospora… The list is long !
In addition to changes at the time of reproduction, fungi can also take on multiple appearances depending on environmental conditions or the vegetative stage of their life cycle. These will obviously depend on the species. Box 15 summarizes the common forms found in Eumycota, the two main ones being the unicellular form called yeast, and the multicellular filamentous form called mold. On a petri dish, yeasts will produce colonies resembling bacterial colonies and the molds to discoid thalli, usually with a much higher growth rate. While the colony shape is fairly constant for yeasts, in the case of filamentous fungi, the appearance of the mycelium depends greatly on the composition of the medium or the temperature (Figure 110). While many species only live in the yeast form and others in the mycelial form, so-called “dimorphic” fungi can alternate between the two. The yeast/hypha and hypha/yeast transitions are under a double genetic and environmental control, which makes their determinism complex. Dimorphic species modulate or are modulated by particular aspects of the fungus’ life, such as the acquisition of virulence in parasitic species or plasmogamy during sexual reproduction. From an evolutionary point of view, it is very difficult to trace the evolution of the different fungal forms. The dispersed phylogenetic distribution of uniquely yeast-like or uniquely filamentous species suggests that they are derived from organisms that originally could do both. Note that the majority of filamentous species at some point in their life cycle make single-celled spores equivalent to yeasts but generally giving rise to hyphae when they germinate. It therefore seems that yeasts evolved from more complex forms by regressive evolution. This hypothesis is supported by recent genomic analyzes which show that filamentous fungi with twice as many genes as yeasts (~ 10,000 versus ~ 5,000), have many genes in common with Metazoa. Such genes appear to have been lost in yeasts.
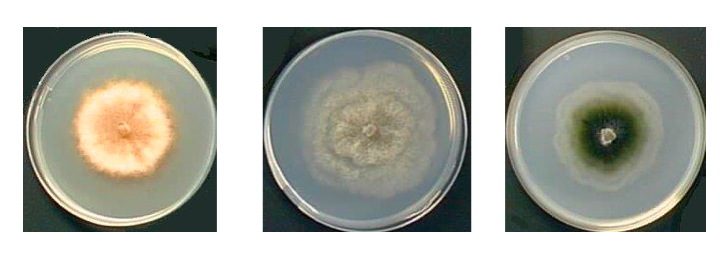
Figure 110.
Podospora anserina grown on three different media. The morphology of the mycelium strongly depends on the culture medium. Left on medium containing ammonium acetate; in the medium on minimum medium containing dextrins and urea; right on a corn decoction.Box 15. Fungal cells
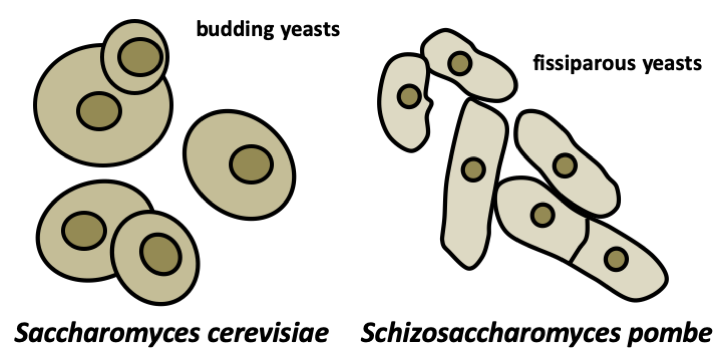
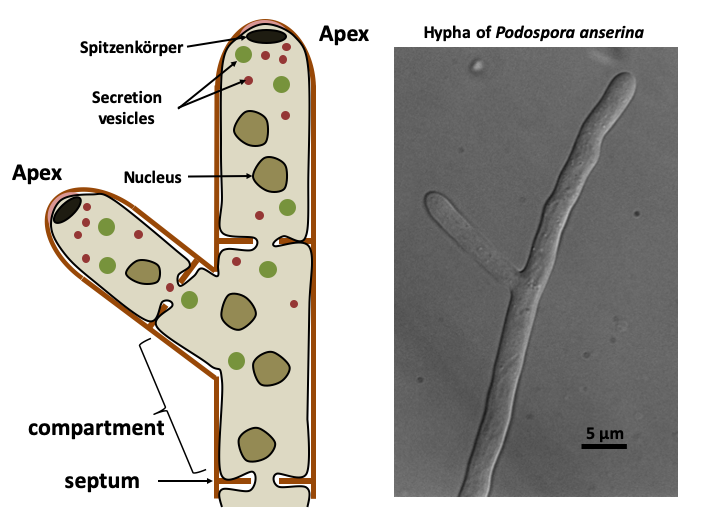
The Eumycota differentiate a variety of thalli depending on their lifestyle. The unicellular yeast form probably provides an advantage for growth in environments where the osmotic pressure is high (like a medium rich in sugar, e.g. fruits) because this decreases the surface area of the organism and facilitates the regulation of the expression of genes. The mycelial mold form is the most common in nature. The mycelium is a collection of hyphae. The hypha is made up of aligned plurinucleated cells, often separated by a septum (pl: septa or septae). Indeed, in Eumycota, the apical cell compartment can separate from the rest of the mycelium by a septum (septate mycelium) or remain in contact with the rest of the thallus (siphoned mycelium). We can think of a hypha as a strained plasmodium in a network of tubing. Growth of the hypha is restricted to the apex. Strictly speaking, therefore, there is no cell division, but rather an extension followed eventually by the formation of septa. The extension is led by the spitzenkörper, a structure made up of a network of actin and vesicles. This one seems to have several roles. It strengthens the apex which is almost devoid of cell wall and prevents the bursting of the cell during hypo-osmotic stress. It additionally produces a pressure which allows the advance of the hyphae and the penetration of the substrate. It would therefore contain a polarisome, a structure that allows polarized growth. If the substrate is too hard, enzymes are excreted to soften it. The spitzenkörper would also serve as a platform for secretion of the enzymes. A spatial decoupling of secretion activity and polarized growth has been demonstrated, showing the complexity of the spitzenkörper. The fungi can thus penetrate the hardest materials. The growth of an apex is actually sustained by several sections which are upstream in the same hyphae and which provide an abundant flow of nutrients, energy, and sometimes possibly nuclei. The compounds arrive in granules visible under a microscope. There are two classes of such granules: 20-80 nm and 80-200 nm in size. They derive from the reticulum via the Golgi apparatus. These granules also ensure the transport of products which are excreted at the apex at the level of the spitzenkörper. In some fungi, they can pass from one hyphal compartment to another because the septae which separate the compartments are pierced with a pore allowing cellular exchanges. The presence and nature of these pores are specific to each group of Eumycota.
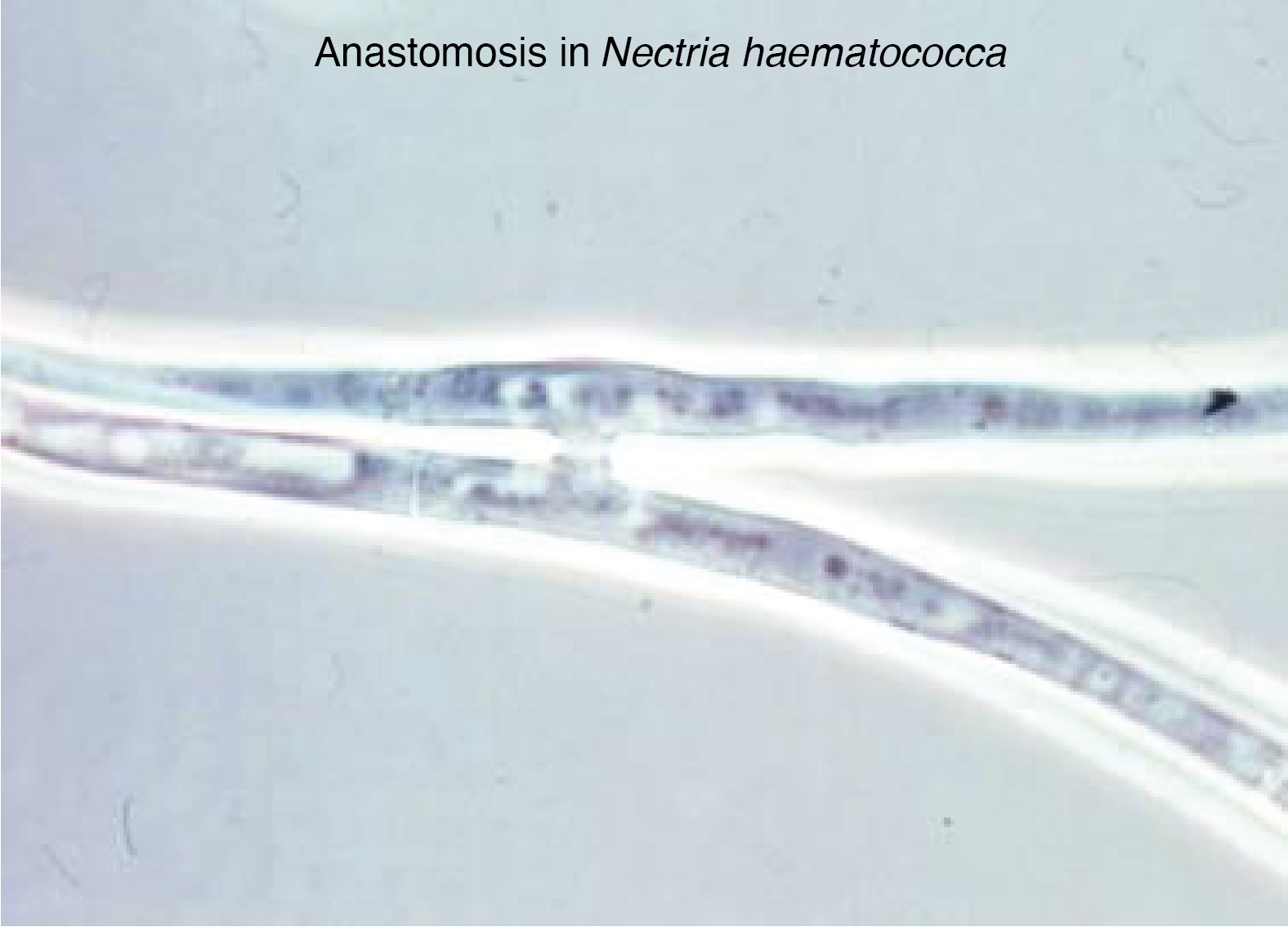
Many branchings are made on hyphae a little further back from the growth front because there seems to be competition at the apex and the formation of branching. Their modalities partly determine the macroscopic morphology of the thallus. The branching is controlled genetically and by nutritional conditions. Another property of hyphae is the possibility in some lineages of making vegetative fusions called anastomoses. At the apex, the hyphae grow away from each other, with anastomoses only occurring when they become older. Further back, the hyphae vacuolate and for the most part slow down growth due to nutritional deficiency. However, these cells remain alive for a very long time, up to several years for some of them! Likewise, for a given species, there are probably several distinct types of hyphae that can be recognized by their diameter. Large hyphae are likely to participate in long-distance transport, while the small ones specialize in exploring the substrate. The cellular form of hyphae allows the development of a mycelium that allows the fungus to invade solid environments in three dimensions very quickly and very efficiently. This is particularly true in the non-homogeneous natural environments encountered by the majority of fungi during their growth. This type of organization in particular allows a different use of the medium compared to yeast colonies (or bacterial colonies). This is because the cells of the mycelial colony that divide always have nutrients available to them. This organization allows staggering extension speeds on Petri dishes: 7 mm/d for Podospora anserina, 3 cm/d for Sordaria macrospora and up to 9 cm/d for Neurospora crassa!
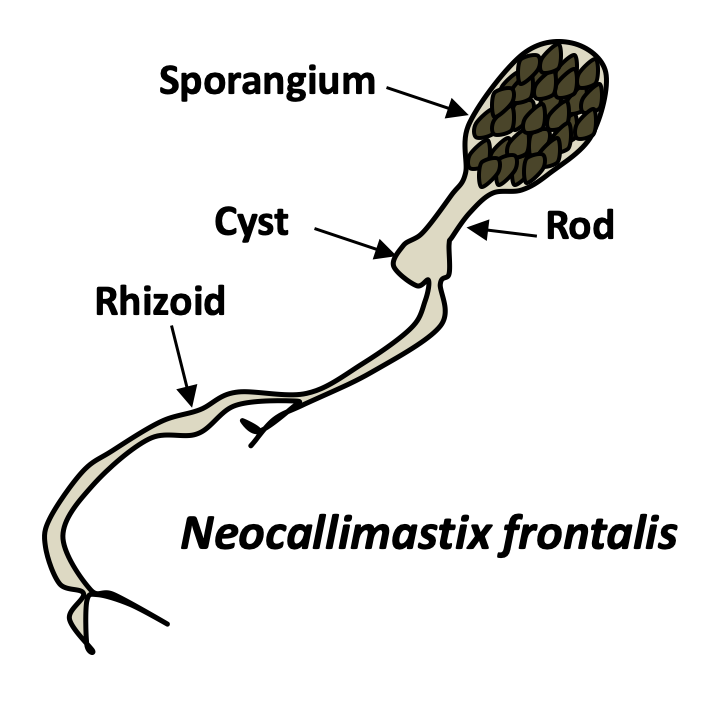
In addition to yeasts and mycelia, Eumycota differentiate thalli of varying shapes. In Chytridiomycota and Neocallimastigomycota, thalli with rhizoids are common. Rhizoids are extensions of the cell in the form of more or less complex ramifications and which improve the penetration of the substrate. The cell usually remains uninucleated, until differentiation of the sporangia containing the spores. Rhizoids are therefore not hyphae even though they perform a similar function. Many animal parasitic species, such as certain Entomophthoromycota or Pneumocystidiomycetes generally do not have a cell wall during their infectious phase. They therefore appear in the form of small plasmodia called protoplasts. This form probably allows the cells to escape the innate immune system, which recognizes cell wall components such as β-glucans. Protoplasts, called mycosomes, are thought to allow the survival of most endophytic species. Found inside plant cells, mycosomes appear to be associated with plastids. Hyphae can assume non-canonical forms because fungi are no less capable of producing highly differentiated cells than animals and plants. Indeed, there is a wide variety of modified hyphae that occur at different times of the life cycle: appressorium of phytopathogenic fungi allowing the penetration of the host, haustorium of lichens and other mutualists, hyphopodium which are used to pump nutrients, lassos of certain nematophagous fungi... For example, in Laboulbeniomycetes, living fungi attach to the cuticle of an insect, the thallus has a fixed number of uninucleated cells with well-defined sizes and shapes. These are involved in nutrition or transmission from one insect to another. Note that the fruiting bodies also have modified hyphae such as cystids, pseudocystids, paraphyses, pseudophyses...
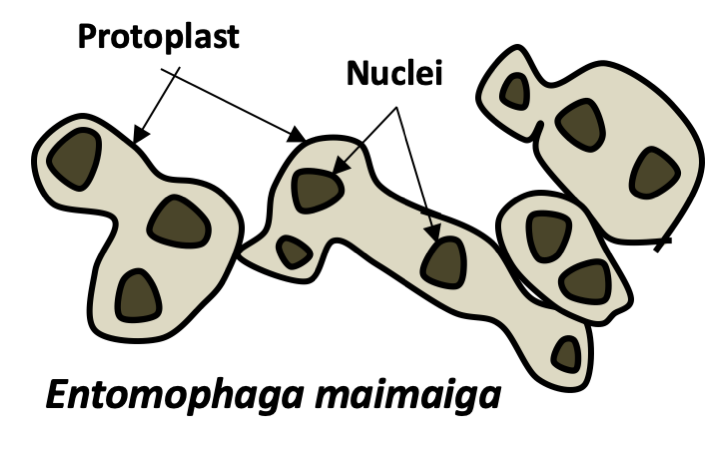
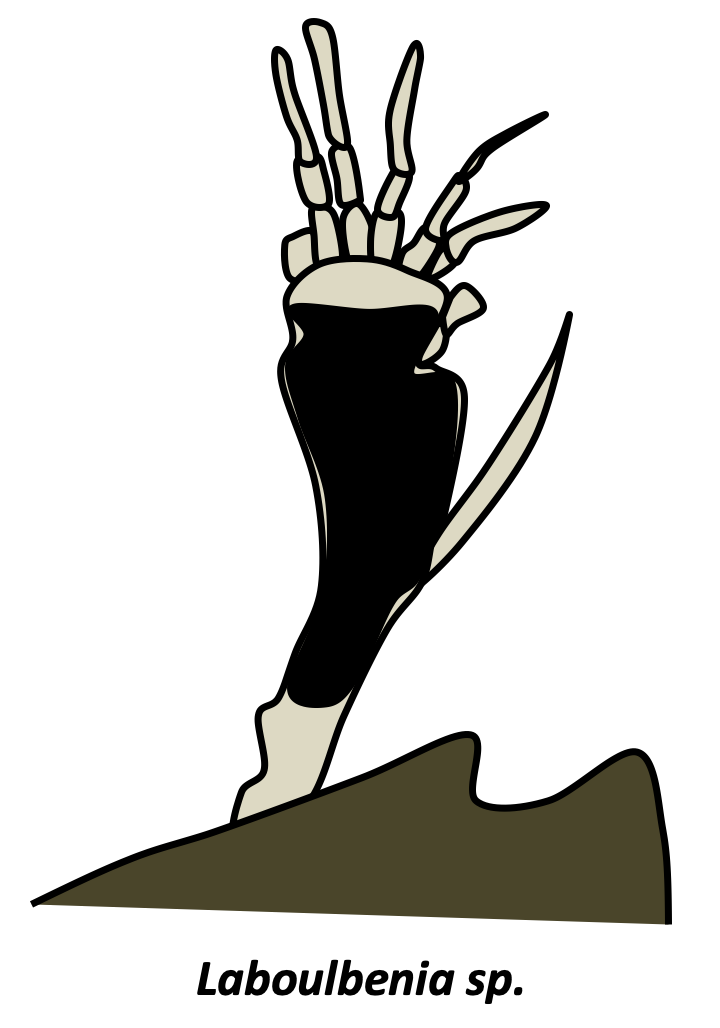
The evolution of Eumycota has long been obscured by the many morphological and biological convergences. Fungi have long been classified as plants. Note that there are still a few researchers that argue that these organisms derive from the Rhodophyta (red algae)! Molecular phylogenies have greatly clarified the phylogeny. They clearly showed that Eumycota are very different from Agaricomycotina and that they are more closely related to Metazoa than Archaeplastida. However, in spite of intense research, knowledge of their evolution still remains incomplete (Figure 111). In particular, as in most lineages resulting from fast evolutionary radiation, the order of branching of the different groups located at the base of the tree varies depending on the genes used to build the tree.
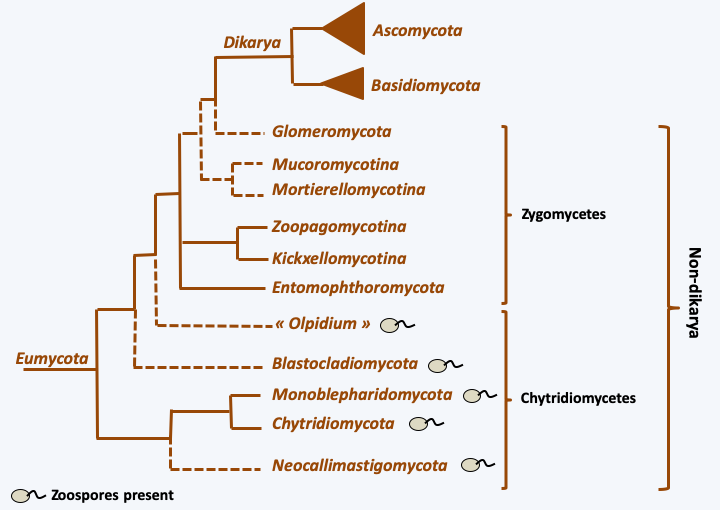
Figure 111.
Phylogeny of Eumycota.Due to the small size of their genomes, Eumycota serve as models for understanding evolutionary mechanisms at the level of complete genomes. Many Eumycota genomes have been sequenced, including the first for a eukaryote, that of the baker’s yeast Saccharomyces cerevisiae. Currently, half of eukaryotic genome sequences available are those of Eumycota. Despite this profusion, the phylogeny remains imprecise for the basal branches. Note thatFigure 111only mentions phylum, without mentioning classes or orders, as there are more than 40 classes and more than 150 orders of Eumycota. Molecular analyzes show that an order represents genetic diversity at least as great as a superclass or even a phylum of Metazoa. For example, the order Saccharomycetales includes organisms as diverse as Yarrowia, Candida and Saccharomyces. The average percentage identity (Figure 112) between the proteins of Yarrowia and those of Saccharomyces is of the same order of magnitude as that between the proteins of Ciona, a Urochordata, and those of humans, a Chordata. There are Saccharomycetales which could be even more divergent… This great dissimilarity is also present at the genomic level, because the synteny of the genes is not very conserved and the gene content is very different between Yarrowia and Saccharomyces. The same diversity has been shown in the Eurotiales or even the Sordariales, which are the two orders of Eumycota that are the most studied apart from Saccharomycetales, showing that the Saccharomycetales are not an exception. Genomic analyzes therefore show that Eumycota have a long evolutionary history because it seems to occur on a longer timescale than that of Metazoa. Animals seem to have appeared 800 million years ago and the Eumycota more than a billion years ago. Their long evolutionary history has thus generated gigantic genetic diversity: there are less than 10 superclasses of animals to compare with the 150 orders of Eumycota!
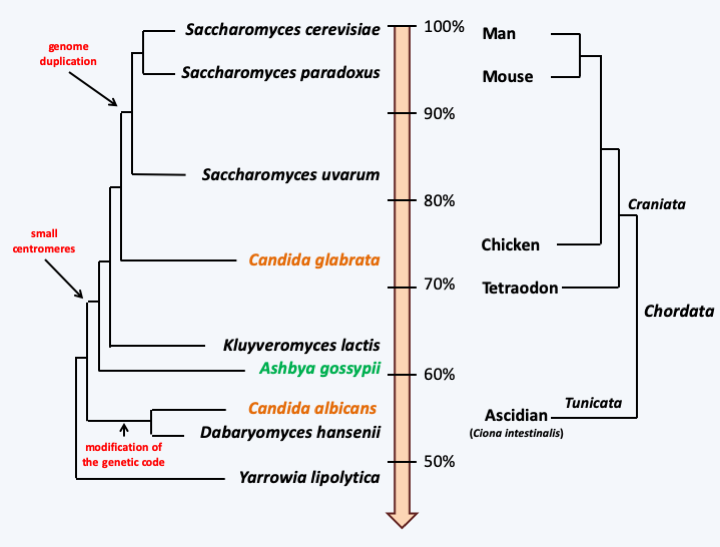
Figure 112.
Comparative evolutionary history of Saccharomycetales and Chordata, based on percentage sequence identity between orthologous genes. In black saprotrophs, in green a plant pathogen and in orange commensals/pathogens of mammals. Only a few major genomic events in the history of Saccharomycetales are indicated.The date given above for the appearance of Eumycota was estimated by an uncertain molecular clock, because the first proven fossils date from the Devonian (around 400 Ma ago) with a predominance of species that are considered to be primitive because they do not present all the characteristics of the two groups considered to be the most complex: the Ascomycota and the Basidiomycota. However, complex Ascomycota fossils dating from this time have been found, showing that the fungi already had a long evolutionary history. The molecular phylogenies confirm multiple events of convergence during the evolution of Eumycota. This is true at the morphological level, in particular at the level of the development of multicellular fruiting bodies (Box 14), but also at the level of nutritional strategies and lifestyles (Box 13). These analyses make it possible to trace an evolutionary pattern (Figure 113). From the ancestral flagellate form, there has been a transition to an osmotrophic diet, accompanied by loss of phagotrophy and acquisition of a cell wall. Note that this ancestral form was also likely to have been able to differentiate into an amoeba form. The reasons for this transition are still unknown. This transition gave rise to aquatic species dispersing using zoospores (flagellated spores), which differentiated from more or less complex vegetative thalli. Soon thereafter, the mycelial form appeared. Indeed, in order to penetrate the solid plant matter more effectively, the Eumycota have independently acquired the filamentous hyphal form several times, initially without septa and then as septate hyphae.
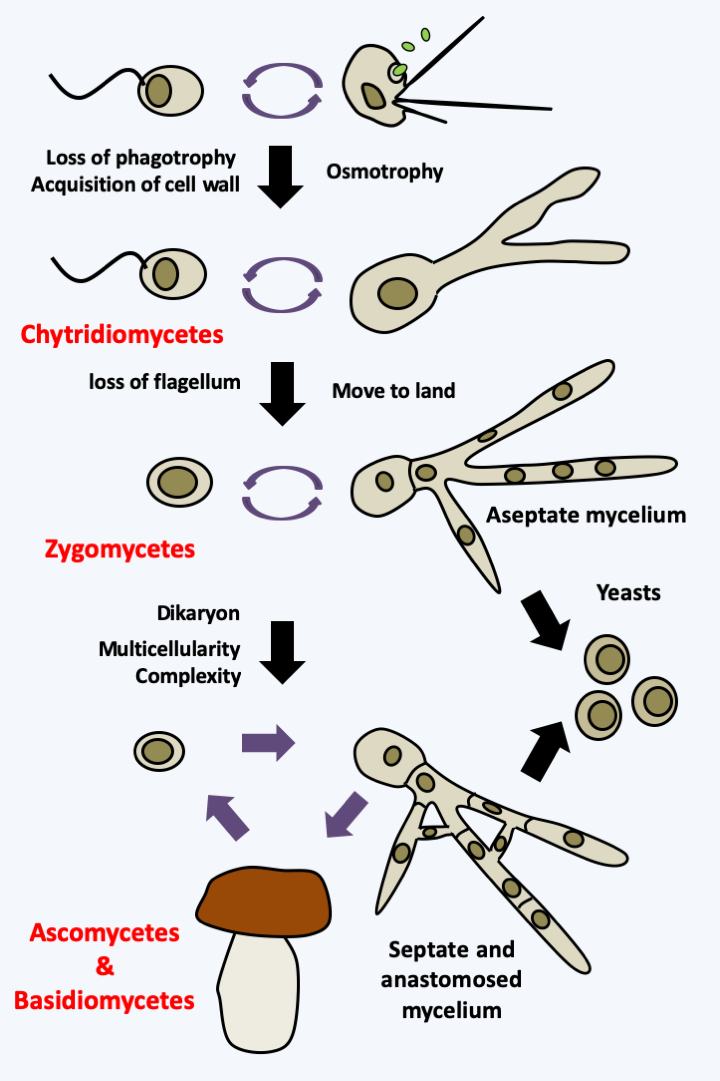
Figure 113.
Evolutionary scheme of Eumycota.Descendants of ancient aquatic fungi retaining a similar lifestyle are still present today. They were historically classified in the old group of “chytridiomycetes” and are now distributed in four phyla (Chytridiomycota, Neocallimastigomycota, Monoblepharidomycota and Blastocladiomycota) and the lineage of Olpidium (Figure 111). Some Eumycota escaped their watery habitats, leading to the loss of the flagellum, which was no longer needed for dispersal in the air. The number of exits from the water is currently under debate. The phylogeny ofFigure 111suggests a single event, but the position of the Olpidium is still under debate. Certain phylogenies place them within terrestrial Eumycota, suggesting in this case at least two independent exits.
The earliest terrestrial Eumycota probably did not differentiate complex mycelia. Indeed, there are currently fungi whose hyphae are still siphoned (without septa) or with complete septa, preventing any transfer of nutrients or information over long distances. Their hyphae usually do not coalesce by anastomosis. Correspondingly, these fungi generally do not make complex sporophores and do not degrade lignocellulose very efficiently, although they do degrade cellulose. On the other hand, certain species have the possibility of adopting a yeast form. Some of these were historically placed in the class of “zygomycetes”. Modern phylogenies classify them into two phyla (Entomophthoromycota and Glomeromycota) and four subphyla (Kickxellomycotina, Mortierellomycotina, Mucoromycotina and Zoopagomycotina), but for the moment they do not allow us to decide whether these lineages form a monophyletic group, or if they are paraphyletic as shown inFigure 111.
A monophyletic lineage, that of Dikarya, presents a mycelium with complex pores between cells in addition to frequent and regulated anastomoses. These characteristics are accompanied by an increased ability to degrade plant material and the production of large multicellular sporophores, especially at the time of sexual reproduction, facilitating dispersal in the air. An important characteristic of this lineage is the acquisition of the dikaryotic state which gives them their name. In fact, in the majority of species, karyogamy is decoupled from plasmogamy. Dikarya therefore generally have a long stage in their cycle in the form of dikaryons with two genetically different nuclei. The selective advantage of this step is not yet clearly understood, but, at least in Basidiomycota, the dikaryon is more competitive than the monokaryons, probably because it allows, as in diploids, genetic complementations between the two genomes present within the same cell. Molecular phylogenies (Figure 111) confirm the 19th century classification of “Ascomycetes” (now Ascomycota) and “Basidiomycetes” (now Basidiomycota). It is in these two groups that the “mushrooms” known to the general public are found. They are often referred to as “higher fungi” in opposition to chytridiomycetes and zygomycetes which are referred to as “lower fungi”, though importantly, these terms are problematic since they suggest different levels of evolution - and all extant species have evolved for exactly the same period of time! Note that many lineages in different fungal groups have undergone evolution for reduced complexity, going from mycelial to yeast forms, and that there are currently zygomycetous yeasts, Ascomycota yeasts and Basidiomycota yeasts.
Zoosporic fungi
Among the five lineages of chytridiomycetes (Figure 111), only two differentiate a mycelium: Monoblepharidomycota and Blastocladiomycota. The other three produce single thalli with or without rhizoids (Figure 114). Despite significant similarities in vegetative thalli, mycologists have long differentiated between different groups of chytidriomycetes based primarily on the ultra-structure of the zoospore. These analyzes show in particular that the flagellar transition zone strongly resembles that found in Choanoflagellata, reinforcing the molecular phylogenies which group Eumycota and Holozoa within Opisthokonta. The reserves that allow the zoospore to move despite the lack of absorption of food are stored in the form of large fat granules positioned in specific places in the cell. The zoospore moves mainly thanks to its flagellum, but in some species it is also capable of amoeboid deformations. Although generally aquatic, some species are adapted to terrestrial life. Indeed, some species belonging to different phyla have lost the ability to differentiate zoospores and therefore only produce aplanospores.
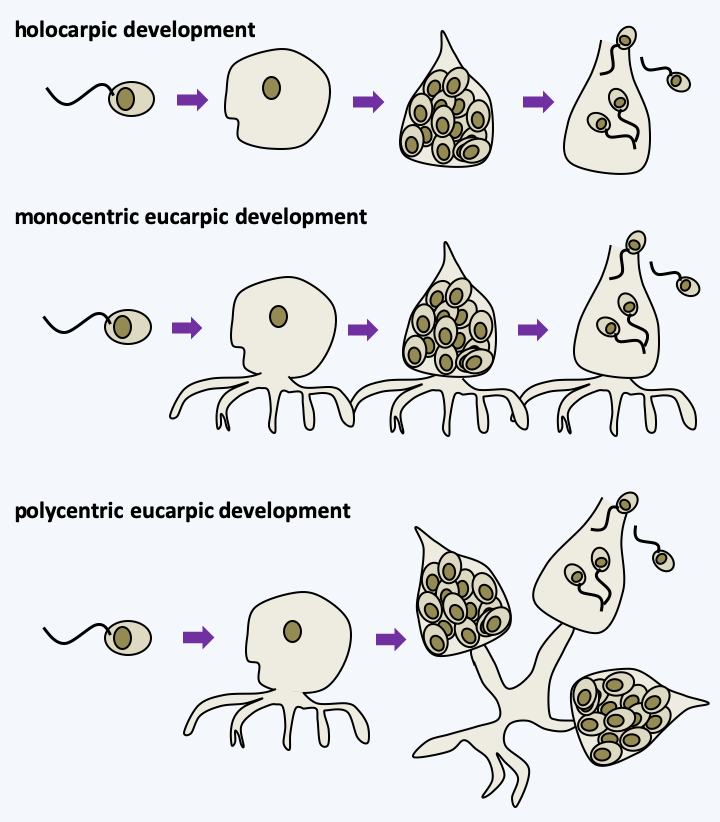
Figure 114.
Types of development in chytridiomycetes. In holocarpic development, the entire thallus turns into zoospores. In eucarpic development, only part of the thallus transforms into zoospores with one or more centers of formation; with respect to monocentric and polycentric thalli, respectively.Recent phylogenies support monophyly between the phyla Chytridiomycota, Monoblepharidomycota and Neocallimastigomycota (Figure 111). Note that different authors sometimes give these lineages different taxonomic rank.
Chytridiomycota
The Chytridiomycota are the most abundant chytridiomycetes. They also have the largest number of described species: a few hundred. They are saprotrophs, parasites of plants, algae, cyanobacteria or animals. The full life cycle is only known for a few species, including Synchytrium endobioticum, a pest of potatoes (Figure 115). This species is able to make a haplobiontic type of sexual cycle, and an asexual cycle. In Eumycota, the haplobiontic cycle is a general rule that has few exceptions. Perhaps the most iconic Chytridiomycota species is Batrachochytrium dendrobatidis, a formidable parasite of frogs responsible for their decline in much of the world. The tadpoles appear to be immune, but an infected adult dies within 15 days, possibly because the fungus disrupts the permeability of the skin. A second related species, Batrachochytrium salamandrivorans, attacks salamanders. In these two species, only the asexual cycle is known. The Chytridiomycota species used as a laboratory model is Spizellomyces punctatus, a monocentric eucarpic saprophyte (Figure 116). Until recently the ecological importance of Chytridiomycota was underestimated. We now know that they are major players in regulating algal blooms and that they are abundant in inhospitable biotopes, such as deep marine sediments and cold regions including tundra, glacial lakes or snowy altitudes.
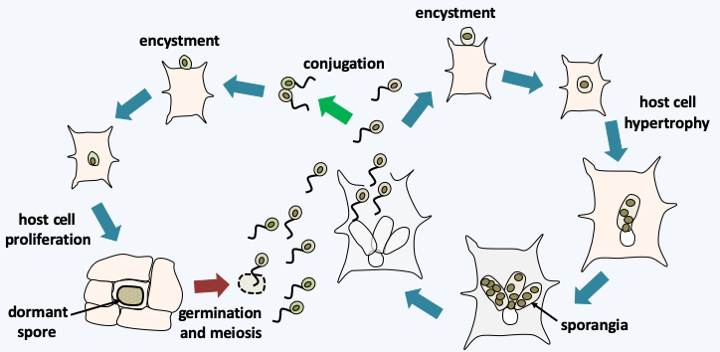
Figure 115.
Synchytrium endobioticum life cycle. This species exhibits a cycle of asexual reproduction which begins with the encystment of a zoospore in contact with a potato cell. After entering the host cell, the fungus proliferates as a plurilobed protoplast, causing the potato cell to hypertrophy. Once ripe, it turns into sporangia, where zoospores ripen. The sexual cycle begins with fertilization as a conjugation between two zoospores. The zygote encysts and then enters the host cell as a protoplast, causing it to proliferate (hyperplasia). This is when the potato grows the warts characteristic of the disease, hence called 'potato wart scab'. Eventually, the intracellular protoplast differentiates into a dormant spore. This eventually germinates to give a sporangium in which meiosis and zoospore differentiation takes place.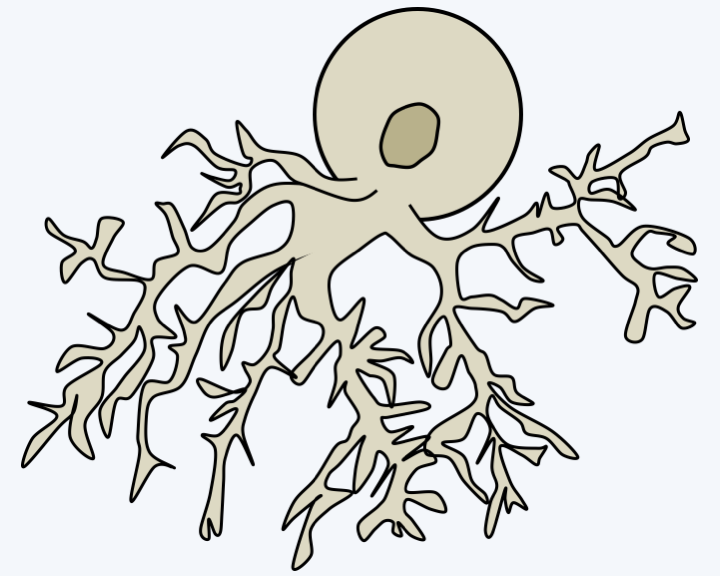
Figure 116.
Thallus of Spitzellomyces punctatus.Monoblepharidomycota
The branch of Monoblepharidomycota has about twenty species that are all saprotrophic. These fungi are distinguished by having polycentric filamentous thalli with a few complete septa. Their mode of reproduction is unique for Eumycota because it involves an “egg”, as in Oomycota which are phylogenetically very distant. This convergence has long intrigued mycologists because in Oomycota the cycle is diplobiontic, while in Monoblepharidomycota it is haplobiontic (Figure 117). These fungi have no other peculiarities that may be of interest to human industry, and their ecological roles are still poorly understood.
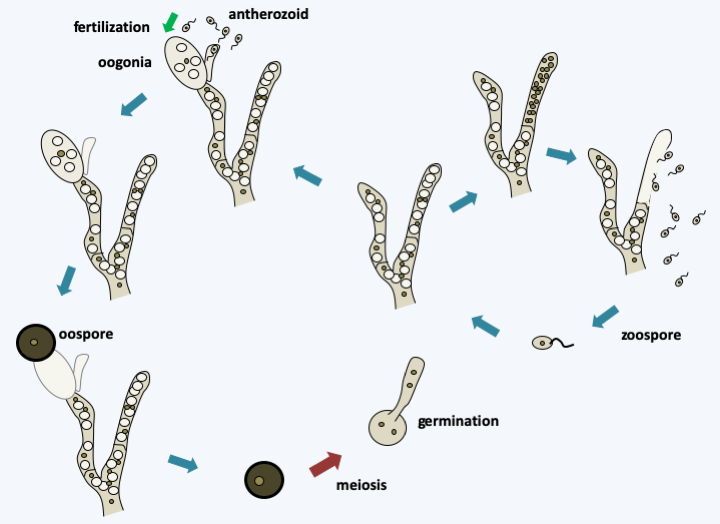
Figure 117.
Life cycle of Monoblepharis polymorpha. Germination of an oospore or zoospore gives rise to a polycentric haploid thallus which can engage in asexual reproduction with the production of zoospores in sporangia. Alternatively, the hermaphrodite thallus can differentiate between male and female gametes. Female gametes are called oogonia because they are large uninucleated cells. Male gametes or anterozoids are small uniflagellate cells produced from a special structure, the antheridium. Plasmogamy is immediately followed by karyogamy and the zygote formed immediately differentiates into a resistance oospore which will allow it to persist during the bad season. This undergoes meiosis and germinates to restore the filamentous haploid thallus. This usually contains many vacuoles, which gives it a characteristic appearance.Neocallimastigomycota
The phylum Neocallimastigomycota contains about twenty species. These are fungi inhabiting the rumen of ruminants and other herbivorous vertebrates, where, along with bacteria and protozoa, they participate in the degradation of plant matter. They were not discovered until 1974 because the presence during their cycle of the flagellated zoospore has long confused them with flagellated protozoa. Vegetative thalli are monocentric, as in Neocallimastix frontalis (Box 15) or polycentric. Their extensive rhizoids penetrate plant debris and the extracellular enzymes they produce are particularly effective in breaking down cellulose. They are obligate anaerobics and possess hydrogenosomes (Figure 31. The hydrogen released is used by methanogenic bacteria, which thereby produce methane, a major greenhouse gas. They are maintained in the rumen thanks to the zoospores which are attracted to the fragments of newly swallowed plants. However, we do not know how they are transmitted to newborn herbivores. It seems that they can differentiate into forms of resistance that have not yet been observed. Sexual reproduction, if it exists, has not been observed in these fungi either. Their enzymes are of interest to industry and several species, such as Piromyces sp. or Orpinomyces sp., have had their genomes sequenced despite the difficulties in cultivating these species.
Blastocladiomycota
About 200 species constitute the phylum of Blastocladiomycota. They have zoospores with a very characteristic morphology: a crescent-shaped structure surrounds the nucleus, which is clearly visible under an optical microscope. It is also one of the few groups of fungi with haplo-diplobiontic life cycles. Most are saprotrophs, but a few species are parasites of terrestrial or aquatic plants or insects. This is the case of Coelomomyces, parasites which alternate between a haploid phase in a crustacean and a diploid form of the protoplast type in an insect larva, often a mosquito larva. One species, Catenaria allomycis, is a parasite of other Blastocladiomycota. The simplest forms have a monocentric thallus, like species of the genus Blastocladiella, while others have a complex polycentric filamentous thallus like Allomyces. The model species of the group is Allomyces macrogynus which is a saprotroph living on plant or animal debris (Figure 118). Note that fossils of Paleoblastocladia milleri resembling present-day Allomyces have been found in sediments dating back to 550 Ma ago confirming that Blastocladiomycota are ancient Eumycota. Molecular phylogenies find it difficult to position them on the evolutionary tree of fungi. Some place them as a sister group to all Eumycota, which would be consistent with the possession of a haplo-diplobiontic-like life cycle and the presence of cholesterol instead of ergosterol in the membranes, a trait they share with the Chytridiomycota. Others, as shown inFigure 111, put them as a sister group to terrestrial fungi. This position would be in agreement with ultrastructural characteristics, such as the possession of a Golgi apparatus devoid of cisterns; a characteristic which they share with the zygomycetes and Dikarya.
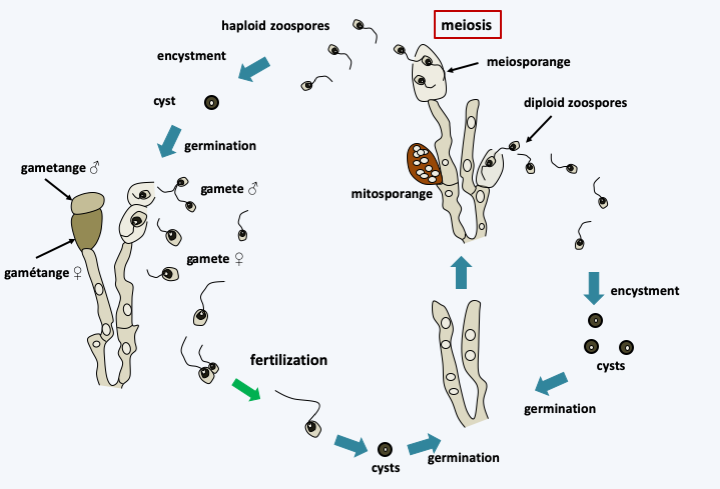
Figure 118.
Life cycle of Allomyces macrogynus. This species has a sexual cycle and an asexual cycle. When the medium is depleted, the diploid mycelium differentiates from sporangia containing zoospores. Zoospores show positive chemotaxis for amino acids. When they reach a suitable place, they encyst and germinate to give back a new mycelium. Diploid mycelium can also produce sporangia with thicker walls in which meiosis occur which result in the formation of haploid zoospores which encyst and give rise to haploid mycelia. When the medium is exhausted, these differentiate at the end of certain hyphae from male and female gametes. Note that the two types of gametes are flagellated but have different size and motility, with the female gamete being defined as the larger and less mobile. In the absence of fertilization, the female gamete can become encysted and give birth to a haploid thallus which will differentiate between male and female gametes. The male gamete is attracted to the female gamete thanks to a pheromone, L-sirenin, which is active at concentrations of the order of 10-10 M. Fertilization gives rise to a mobile uniflagellate zygote which quickly encysts and gives back a diploid thallus.Olpidium
The last group of chytridiomycetes, the Olpidium, are obligate parasites of plants and cannot be cultivated without their hosts. Their life cycle is simple without known sexuality (Figure 119). The phylogenetic position of Olpidium is currently under debate, but recent evidence support Olpidium as the sister group to all terrestrial fungi as shown in Figure 111, suggesting a single flagellum loss when coming out of the water during the evolution of fungi.
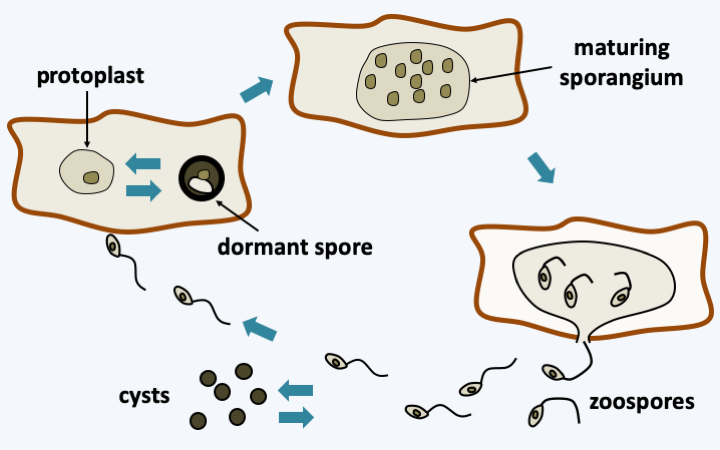
Figure 119.
Olpidium bornovanus life cycle. This species parasitizes cucumber roots. Root cells are dispersed and infected by zoospores. These have the possibility of becoming encysted. Inside the host, the parasite grows monocentrically as a protoplast that will differentiate into a dormant spore or a sporangium. Zoospores are then expelled.Zygomycetous fungi
The other lineages of Eumycota do not produce zoospores. However, in zygomycetes, the organizing center of the microtubule network, called “SPB” for Spindle Pole Body, always have a structure very similar to that of chytridiomycetes, with the presence of microtubules arranged in a bundle, a structure interpreted as the vestige of a centriole. Zygomycetes produce mainly two types of aplanospores. Meiospores are usually the result of the fusion of two dumbbell-shaped hyphae called suspensors. The wall of the meiospores, called zygospores, is very thick and they are used for persistence. Zygomycetes also make mitospores in structures called sporangia. These spores are called sporangiospores or conidia.
Zoopagomycota
Current phylogenies support monophyly of the groups Entomophthoromycotina, Zoopagomycotina and Kickxellomycotina.
Entomophthoromycotina
The Entomophthoromycotina phylum mainly includes the zygomycetes formerly classified in the orders Entomophthorales, Basidiobolales and Conidiobolales. They are characterized by the production of conidia that are actively expelled. These have the possibility of germinating to restore so-called secondary conidia. Entomophthoromycotan genomes appear to be larger than in other Eumycota, with sizes in the order of 700 Mb in Basidiobolus and 8000 Mb in Entomophthora aulicae! Most of the few hundred species in this group parasitize insects, but those that diverged first are saprotrophs or live associated with vertebrates. For example, Basidiobolus ranarum is frequently isolated from frog droppings, but whether or not it persists in the amphibian is not known. Species of the genus Conidiobolus are also saprotrophs capable of parasitizing insects and even humans. Some species produce a mycelium, while other species generally only produce protoplasts in the general cavity of the insects they parasitize. Their haplobiontic cycle may or may not exhibit sexual reproduction (Figure 120). Studies carried out to determine their potential in the fight against insects show convincing results for long-term control of insect populations. However, they cannot curb an epidemic situation.
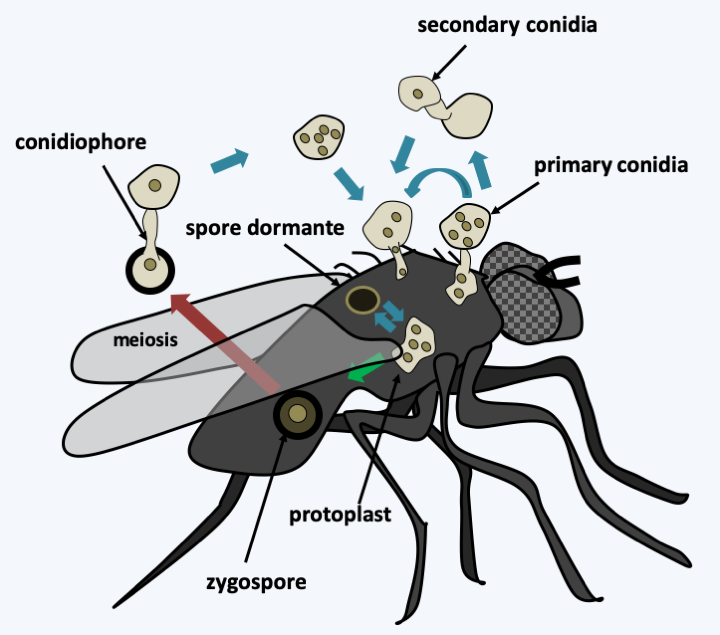
Figure 120.
Entomophthora muscae life cycle. This species parasitizes house flies. After entering the insect via the germination of a conidium, the fungus differentiates into invading protoplasts and then killing the host. It then most often enters the asexual cycle with the production of primary conidia on the surface of the insect. These can directly re-infect new hosts or germinate to give back secondary conidia which appear to be more infectious. The fungus can also more rarely enter the sexual cycle with the production of zygospores. Meiosis probably takes place in the zygospore. This persists in the environment and then germinates to immediately give a conidium which is the infectious propagule. These fungi appear to be homothallic. They also presumably produce dormant spores.Zoopagomycotina
Zoopagomycotina are only known by a few dozen species. They are mainly parasites of other fungi and small protists or animals such as amoeba, rotifers or nematodes that they trap with adhesive globules.Figure 121depicts the life cycle of the type species Piptocephalis fresenia. Little is known about the biology of these fungi.
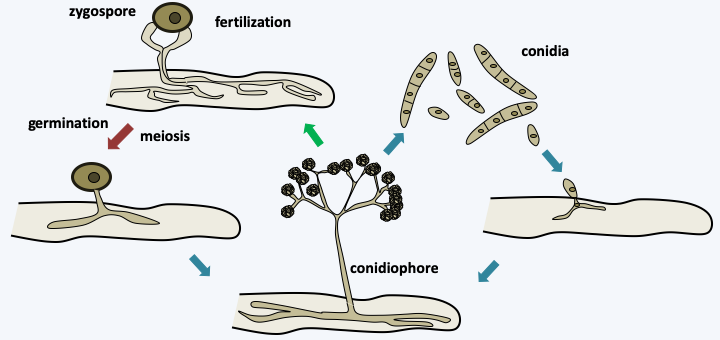
Figure 121.
Life cycle of Piptocephalis fresenia. This parasite attacks Mucorales. It has a haplobiontic cycle typical of Zoopagomycotina. The infectious propagules are mainly conidia. After germination, the germ tube is attracted by the mycelium of its host which is chosen because it has a particular wall structure. After development, the mycelium generates conidiophores characteristic of Piptocephalis. The sexual cycle passes through the formation of a zygospore resulting from the fusion of two compatible hyphae, most species being homothallic. Meiosis takes place in the zygospore probably at the time of germination.Kickxellomycotina
The Kickxellomycotina subphylum has been defined primarily by molecular phylogenies. The species in the group differentiate between hyphae whose cells are separated by partitions pierced with particular pores, the lenticular pores (Figure 122). Apart from this shared character, the different groups of Kickxellomycotina have very variable morphologies and ecologies: Harpellales are parasites of larvae of aquatic insects or crustaceans, Kickxellales are mainly saprotrophs, Dimargaritales parasitize other fungi and Asellariales are parasites or commensals of insects. The thalli can be more or less filamentous and give rise to apparatuses carrying more or less complex spores. Their life cycles are classic with the production of asexual spores, infectious in the case of parasites, and in some species we note the presence of sexual reproduction with the haplobiontic type cycle typical of Eumycota.
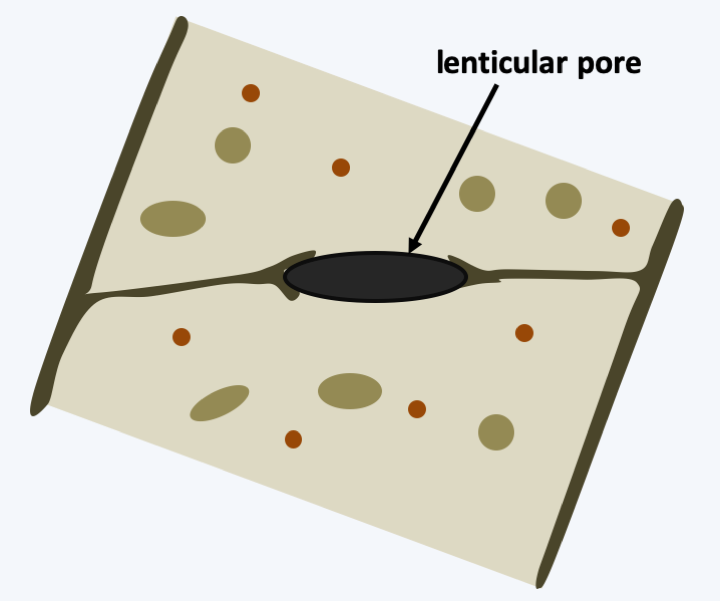
Figure 122.
The lenticular pore of Kickxellomycotina.Mucoromycota
The last three lineages of zygomycetous fungi, Mucoromycotina, Mortierellomycotina, and Glomeromycotina, have many characteristics that make them similar to Dikarya. Moreover, molecular phylogenies place them as monophyletic and as a sister group to the Dikarya.Figure 111gives a phylogeny with “Glomeromycota” as a sister group to Dikarya, with Mortierellomycotina and Mucoromycotina being more distant. Most recent phylogenies, however, instead place the glomeromycetes within Mucoromycota, giving phylum status to Mucoromycota and subphylum status to Glomeromycotina. The three groups are thus probably monophyletic, but the branching order within this monophyletic group is still unresolved. All three of these groups produce extensive mycelia (Figure 123) with hyphae rarely possessing complete septa. The integration of the thallus therefore remains simple. Nevertheless, movement of the cytoplasm ensure communication. In Glomeromycota, the mycelium is able to initiate anastomoses at low frequency; it is not clear whether Mortierellomycotina and Mucoromycotina are capable of doing the same. Likewise, in general, when the mycelium is injured, the cytoplasm becomes very viscous at the point of injury, thus limiting the damage to the thallus. The cell wall of these groups contains, in addition to chitin, chitosan: a deacetylated derivative of chitin. Some Mucoromycotina are able to differentiate yeasts in response to variations in certain environmental stimuli such as temperature or pressure in carbon dioxide or oxygen. These fungi repeatedly evolved multicellular fruiting bodies which, however, remain simple (Figure 124).
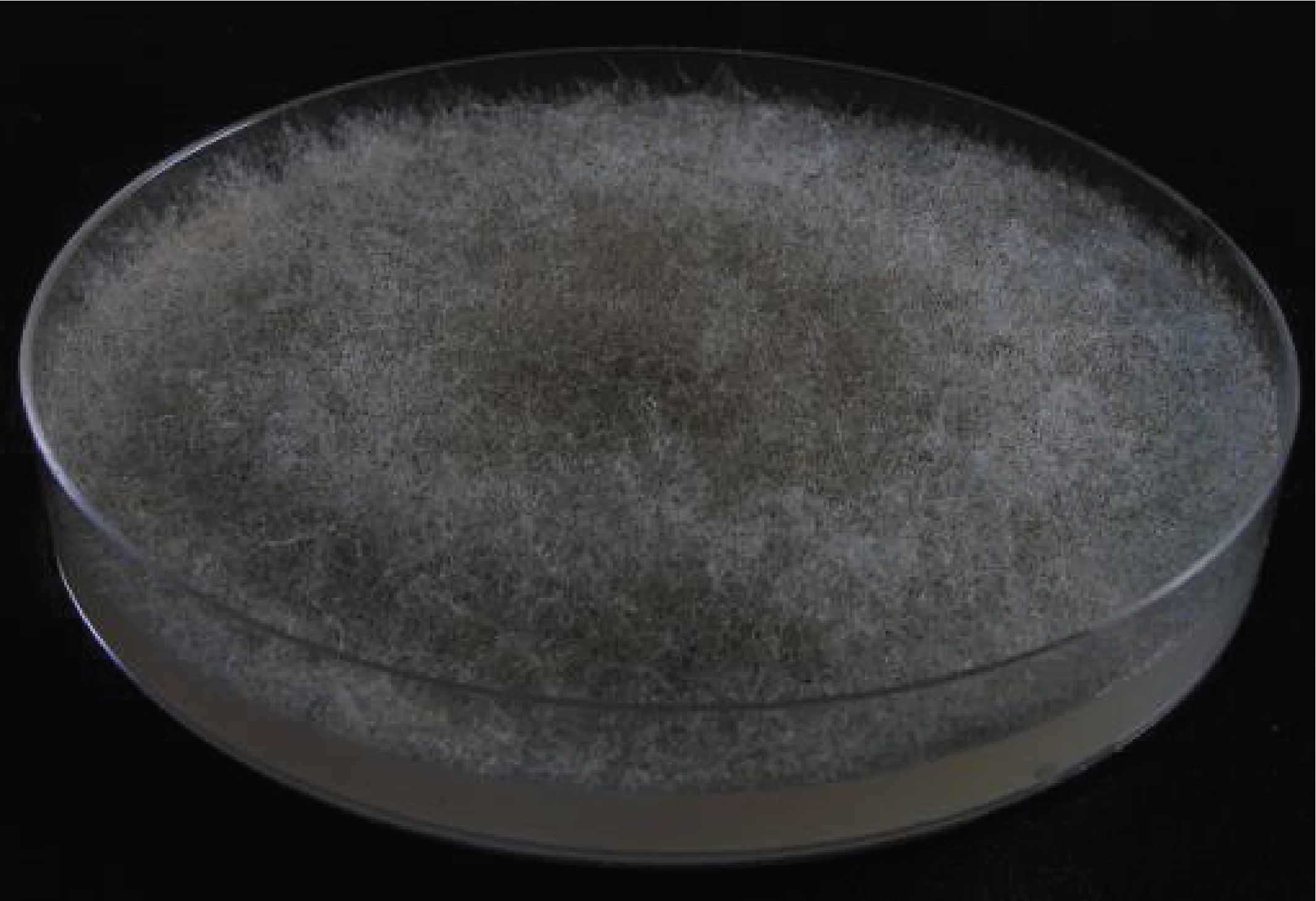
Figure 123.
Mycelium of Rhizopus oryzae.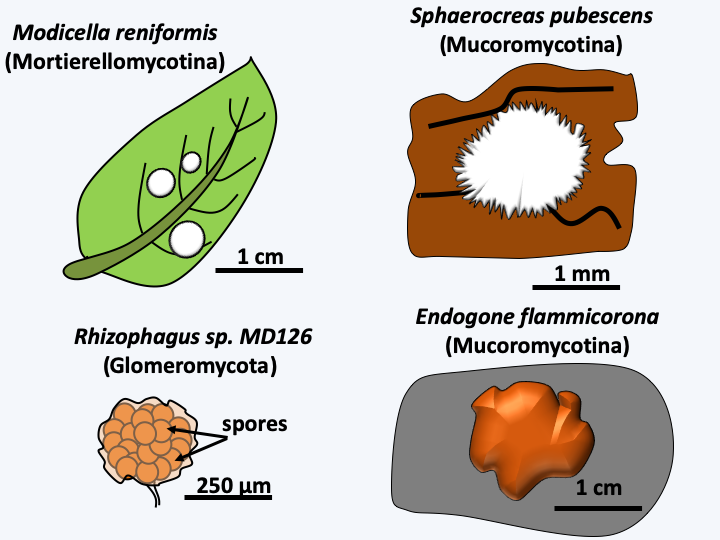
Figure 124.
Multicellular fruiting bodies in zygomycetes. These fruiting bodies are composed of poorly differentiated tissues that contain asexual spores.Mucoromycotina
Mucoromycotina are currently known by a few hundred species, some of which are used in basic research, such as Phycomyces blakesleeanus (Figure 125) or Mucor circinelloides. Most are saprotrophs of soil or herbivorous droppings (Figure 126), but some species of the order Endogonales are endomycorrhizal fungi. Some species such as Rhizopus oryzae (Figure 123) are used in the fermentation and ripening processes of food. Several species, mainly of the order Mucorales, can cause rare but serious yeast infections in humans, called zygomycosis. Most Mucoromycotina have sexual and asexual cycles (Figure 127), but in Endogonales, reproduction does not appear to involve asexual sporulation and relies instead only on the production of zygospores grouped together into a multicellular fruiting body about 1 cm in diameter (Figure 124).
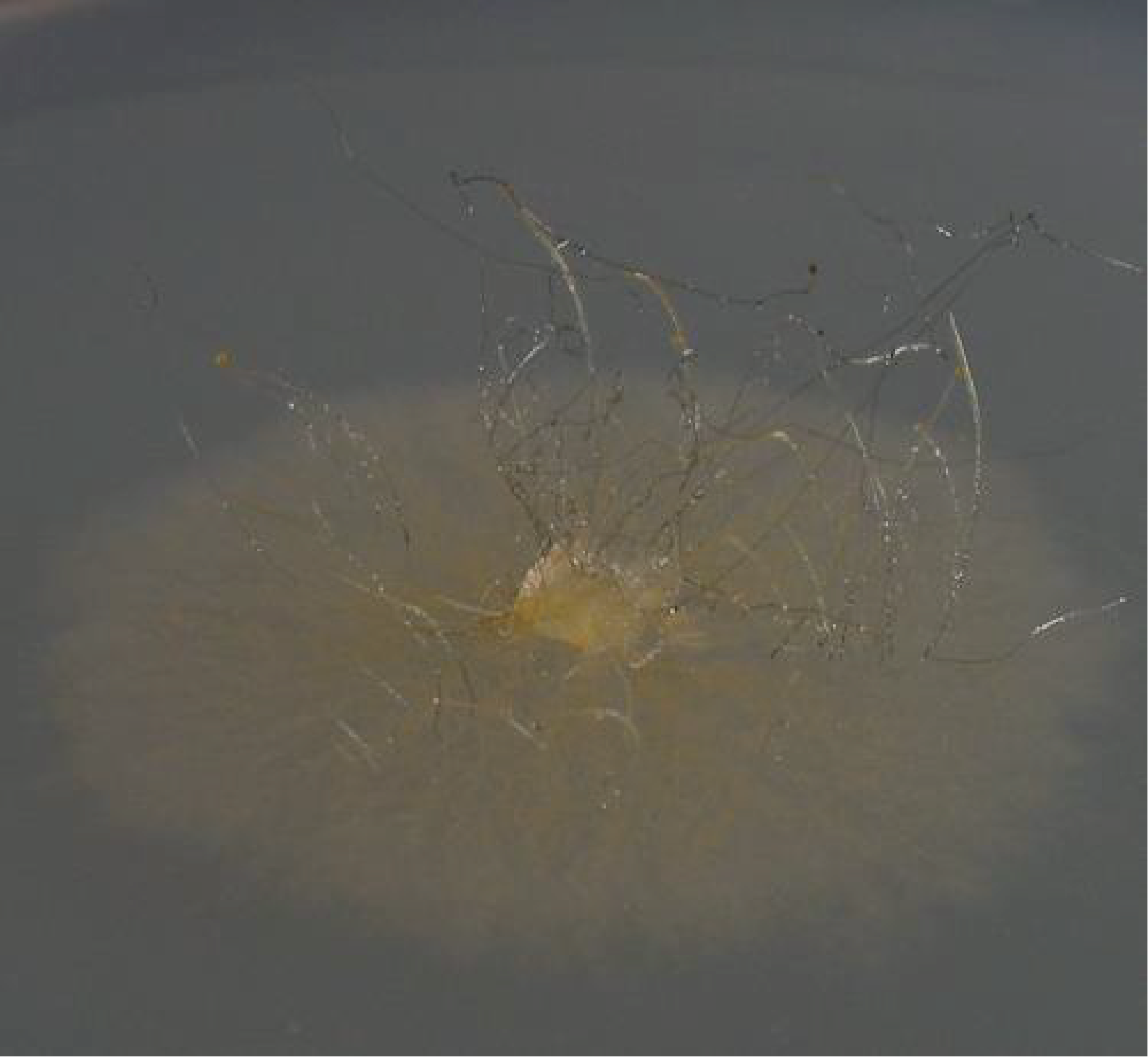
Figure 125.
Phycomyces blakesleeanus, a laboratory model. This Mucoromycotina is one of the most remarkable species. It produces giant sporangiophores 10 to 20 cm high with rapid and controllable growth. In particular, they can be excised and complete their development independently. They have interesting properties which make them good models to study the influence of physical phenomena on morphogenesis. In particular, they have positive phototropism, negative geotropism and more surprisingly they exhibit an avoidance mechanism. Indeed, about three minutes after an object is placed near the sporangiophore, i.e., at a distance of 1-3 mm, the growth of the latter is deflected by about 1° to 2° per minute! The sensory mechanism for this response is unknown.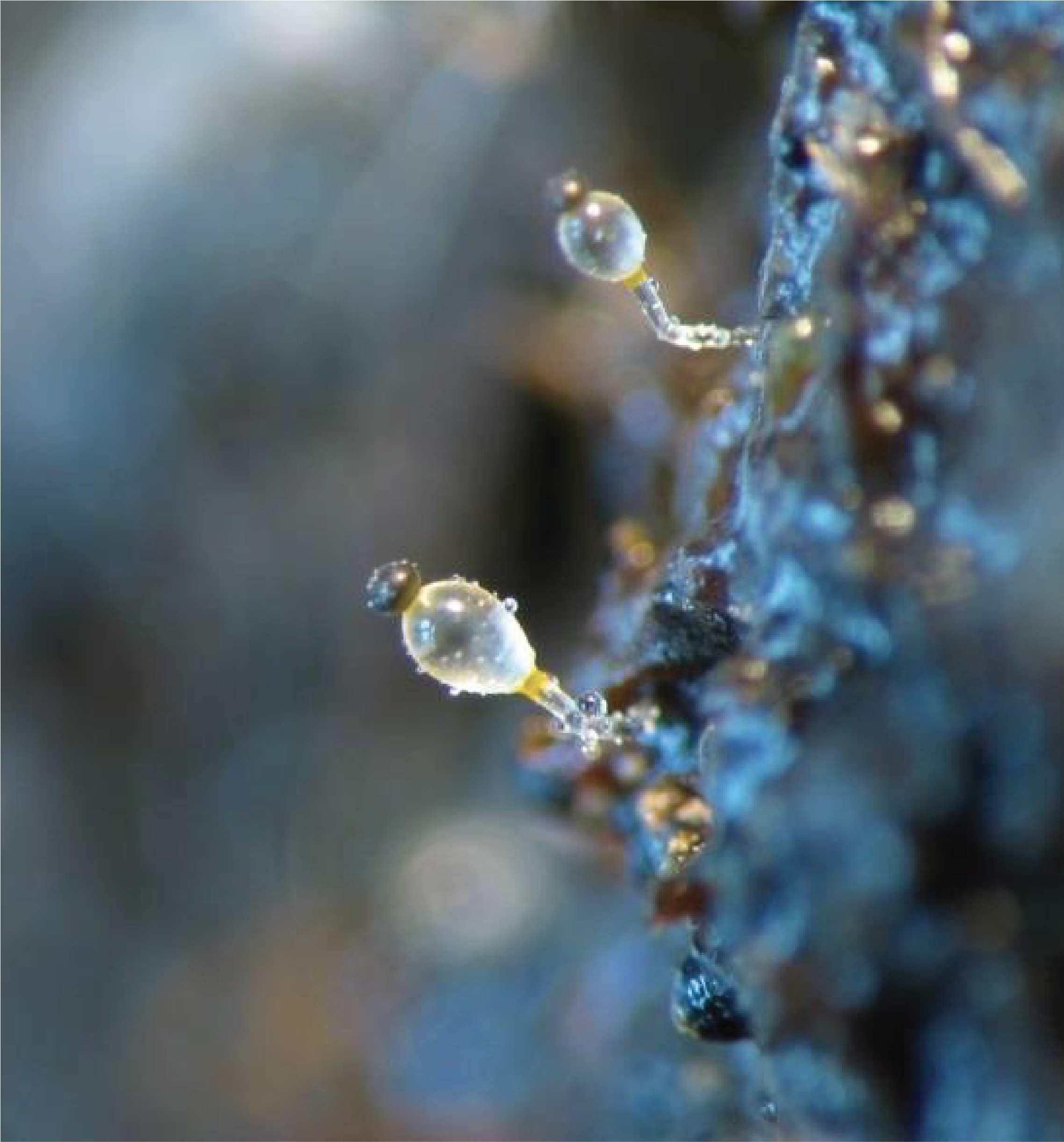
Figure 126.
Pilobolus crystallinus a species dependent on herbivorous excrement. This species reproduces by generating massive sporangiophores carrying at their tip a compact sporangium which is expelled at a distance of several tens of centimeters after the bursting of a characteristic vesicle. The sporangiophore orients itself towards the lumen ensuring efficient ejection of the sporangium. This one is sticky and attaches to surrounding grasses. Its ingestion by a herbivore allows the germination of the spores.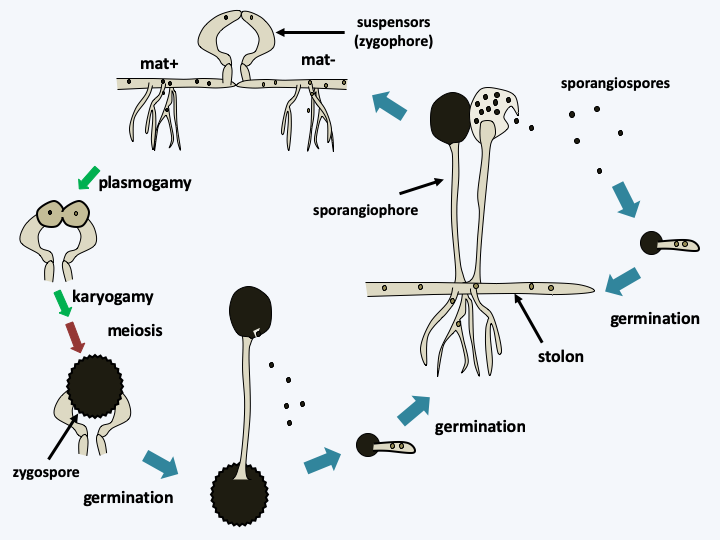
Figure 127.
Life cycle of Mucor mucedo. The cycle begins with the germination of a multinucleated and haploid sporangiospore to give rise to a mycelium made up of different types of hyphae: large-diameter horizontal hyphae that grow rapidly on the surface of the substrate, stolons, and smaller hyphae which penetrate the environment and provide nutrition. The mycelium most often engages in an asexual cycle and quickly differentiates into sporangiophores. In Mucor mucedo, there are two sexual types. Each of the sex types has an incomplete pathway for the biosynthesis of a hormone, trisporic acid. It is only produced when the two mycelia mat+ and mat- are present. When strains of compatible sexual types are found, the mycelia differentiate, under the control of trisporic acid, into zygophores or suspensors. The plurinucleated zygophores grow towards each other and after a complex process of differentiation, they merge to give rise to a zygospore. In this zygospore, the nuclei pair up in pairs and then merge and the diploid nuclei obtained form meiosis. The fate of the different nuclei is not always clear because, depending on the species, a zygospore gives rise to descendants of a single sexual type such as Mucor mucedo, or to descendants of one or the other type. Zygospores germinate and directly produce a sporangiophore. The zygospore is particularly resistant and germinates with a low and constant germination percentage of around 1%. This phenomenon is probably linked to a mechanism of dormancy that is gradually lifted over time.Mortierellomycotina
The Mortierellomycotina subphylum contains about 100 species living mainly as saprotrophs inhabiting the soils. They can also be isolated as endophytes. One species, Mortierella wolfii, can infect cattle. Their life cycle is identical to that of Mucoromycotina. The ability of certain species such as Mortierella alpina or Mortierella isabellina to manufacture large quantities of polyunsaturated fatty acids gives these fungi an interest for the pharmaceutical and agro-food industries.
Glomeromycotina
The Glomeromycotina subphylum contains about a hundred species divided into five orders, the Glomerales, Diversisporales, Archaeosporales, Paraglomerales and the Geosiphonales. Currently the order Geosiphonales contains a single species, Geosiphon pyriformis, which lives in association with cyanobacteria of the genus Nostoc (see Box 10). It is a rare organism that lives among mosses in a mountainous region of Germany where it has only been harvested a few times! The type of association it makes with cyanobacteria is currently unique in Amorphea. The bacteria can live perfectly on their own, but this is not the case with the fungus. As Nostoc performs photosynthesis and can fix atmospheric nitrogen, the union is fully self-sufficient. After the fungal spore germinates, the fungus must find its companion, since the spores are devoid of Nostoc. Cyanobacteria are only competent to form the symbiosis at one point in their development cycle and recognition by the fungus seems specific to certain strains of Nostoc punctiforme. It also seems that for the symbiosis to be successful, a phosphate deficiency is necessary. We do not currently know the entire life cycle and it is possible that Geosiphon pyriformis also engages endomycorrhizal type associations with plants. In fact, molecular phylogenies show that the evolutionary lineage leading to this species is included in that of the Glomales. Associations with cyanobacteria could be earlier than the establishment of mycorrhizae with terrestrial plants by other Glomeromycota.
The remaining Glomeromycotina orders, sometimes referred to as AM (arbuscular mycorrhizal) fungi, are probably monophyletic and much more important from an ecological point of view because they are abundant in terrestrial ecosystems, although they often go unnoticed. Indeed, they form the so-called vesiculo-arbuscular endomycorrhizae with the majority of plants, since about 90% of vascular plant species and many other plants, including some bryophytes, live in association with AM fungi. It has been shown that the presence of these fungi in biotopes not only improves biomass production but also increases plant biodiversity. Although the different morphospecies of AM fungi show host preference, it seems that they are able to associate more or less efficiently with almost any plant! Vesiculo-arbuscular endomycorrhizae owe their name to the presence of lipid vesicles and the shape of intracellular hyphae; a highly branched structure somewhat resembling a tree (arbuscula = tree). The association is essential for the fungus, and it is very difficult, if not impossible, to cultivate them on a Petri dish. It seems that these fungi cannot properly assimilate glucose from the soil and that the elongation of fatty acids is only possible when the fungus is associated with the plant. Their harvest and cultivation can therefore only be done with the partner plant. The life cycle is simple and apparently devoid of sexual reproduction (Figure 128). This last point is still under debate because it seems that rare genetic recombination can occur in certain species. However, since the poorly septate mycelium of Glomeromycotina species is capable of anastomosis, the presence of a parasexual cycle cannot be ruled out. The exact role of anastomoses is still unknown in Glomeromycotina. They could allow the mixing of nuclei of different origins and promote genetic diversity. In contrast, it has been shown that there are incompatibility mechanisms between isolates of the species Glomus mossae, similar to those present in Dikarya, which act by preventing the effective fusions of hyphae; however, the first stage of recognition seems to be happening. This barrier could limit the spread of intrahyphal bacteria present in different isolates, some of which do not appear to be mutualistic. The genetic makeup of AM fungi has long been a subject of debate. Complete genome sequences show that these organisms have mycelia possessing genetically homogeneous nuclei, but exhibiting a strong polymorphism between the units encoding ribosomal RNAs. As these units have been widely used to measure genetic diversity, this has led to an overestimation of the genetic diversity of these fungi. The genomes of AM fungi are unusually large compared to other fungi: over 100 Mb in many species, spread out across more than 30 chromosomes in the model species Rhizophagus irregularis! Note that the morphology of Glomeromycotina spores is characteristic and that Ordovician fossils (400 Ma old) allow their appearance to be dated to the same period as the evolution of terrestrial plants (450 Ma ago). It is possible that Glomeromycota and/or Endogonales could have been instrumental in allowing plants to exit the oceans towards terrestrial environments by facilitating uptake of soil nutrients.
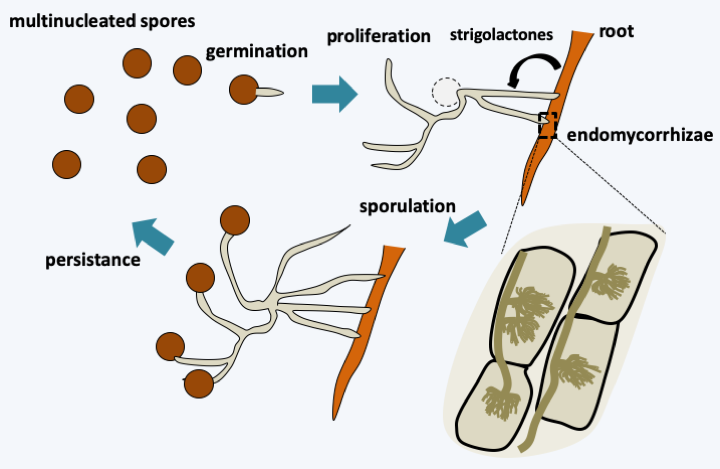
Figure 128.
Rhizophagus irregularis life cycle. Multinucleated spores ensure the persistence of the fungus in the soil. The spores germinate without the presence of a plant. However, the mycelium formed in the absence of a root quickly stops growing and returns to a slower metabolic state of life. If a plant root passes near this mycelium, it branches out extensively and penetrates the root. Communication takes place via the emission by the plant of a strigolactone molecule (5-deoxy-strigol) which acts at very low concentration because 3 μg placed on a disk close to a mycelium are sufficient to induce the proliferation of hyphae. This molecule causes an increase in the mitochondrial metabolism of the fungus, probably allowing an increased metabolism. The activity of the fungus also seems to be controlled by other plant hormones including abscisic acid and ethylene. Endomycorrhizae are formed and are accompanied by the proliferation of extra-root mycelium. The cycle ends with the formation of spores which are very large (just under 1mm in diameter). These often contain more than 1000 nuclei.Dikarya
Despite their great genetic diversity, the fungi discussed so far together represent only about 5% of the described species of Eumycota. In fact, the vast majority of current species belong to the monophyletic line of Dikarya, comprising the two phyla Ascomycota and Basidiomycota (Figure 111andFigure 129). Some lineages of Dikarya, such as Taphrinomycotina and Saccharomycotina in Ascomycota, and Pucciniomycotina and Ustilaginomycotina in Basidiomycota, retain some characteristics of their more distant relatives, in particular minimal thallus integration and the production of multicellular fruiting bodies which are still simple. Nevertheless, in certain cases, a regressive evolution, in particular in Saccharomycotina, seems in part responsible for this simplicity.
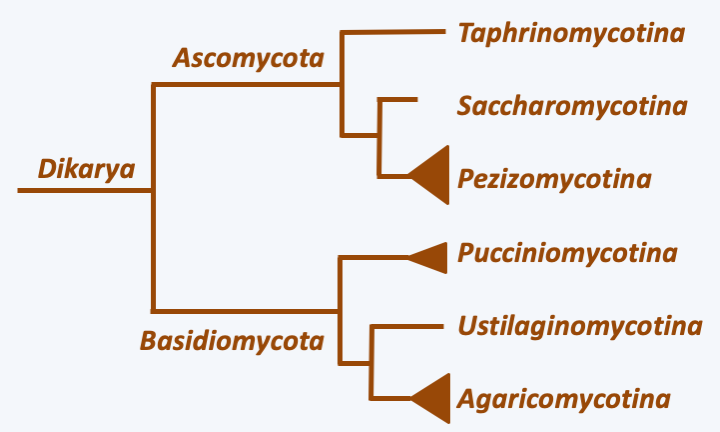
Figure 129.
Phylogeny of Dikarya.In the two most “complex” subphyla, Pezizomycotina in Ascomycota and Agaricomycotina in Basidiomycota, integration of the thallus is maximal and the production of complex multicellular fruiting bodies is the rule. Indeed, in these two groups, the hyphae have partitions pierced with a central pore associated with structures allowing the selective passage of nutrients, organelles and information, promoting intercellular communications at short and long distances. They frequently engage in anastomoses. These occur at different times, in particular during the dikaryotic stage which characterizes the Dikarya cycle (Figure 130), as they participate in maintaining the dikaryotic state (Figure 131). Surprisingly, it seems that this increasingly complex integration of the thallus occurred by convergent evolution. Indeed, the structure of the pores connecting the cells is different in Ascomycota and Basidiomycota. Likewise, the control of the clamp formation of Basidiomycota anastomosis is under the control of the mating receptor/pheromone system and homeodomain transcription factors, while the crozier formation of Pezizomycotina is not.
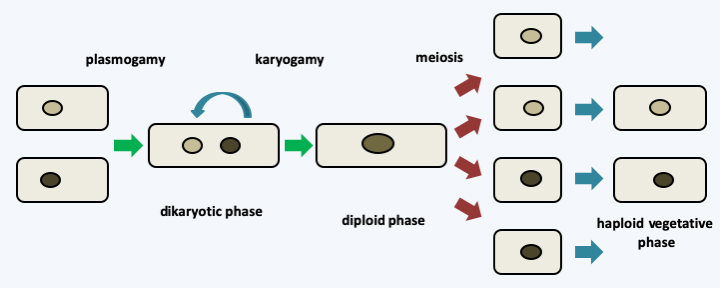
Figure 130.
Typical life cycle of Dikarya. In the Dikarya, the cycle is mainly of the haplobiontic type and meiosis is preceded more or less by a long stage in the form of a dikaryon. Nevertheless, many variations exist such as, for example, haplobiontic or haplodiplobiontic cycles without an extended dikaryotic phase in certain yeasts.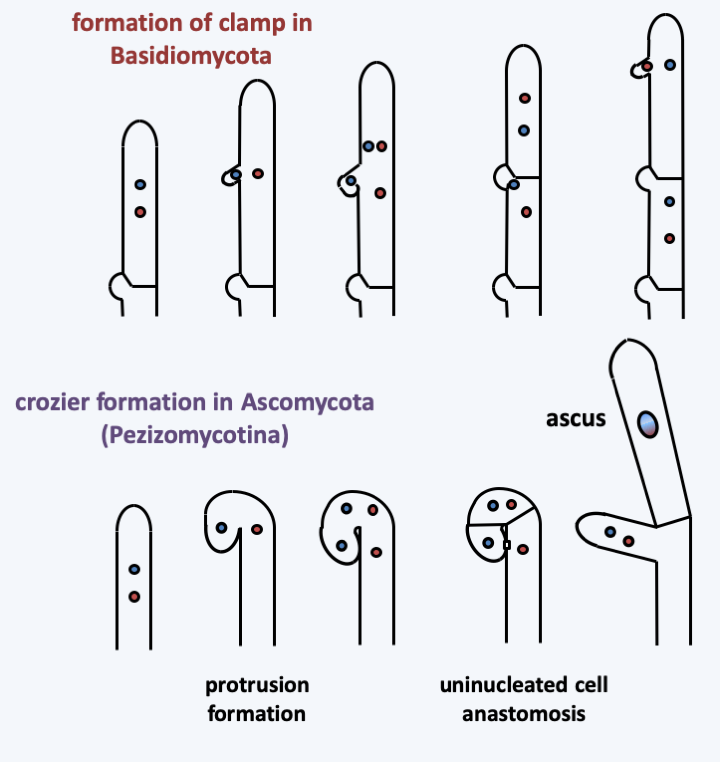
Figure 131.
Mechanisms of dikaryotic state maintenance in Dikarya.The mycelium of Dikarya is in general capable of behaviors that cannot be adopted by those of other fungi. First, they can better explore their growth substrate by differentiating between hyphal bundles that can transport large amounts of metabolites and organelles over long distances. The mycelium can thus pass through large regions devoid of nutrients (Figure 132). Other mechanisms allowing better long-distance communication are suspected such as the emission of small molecules with various properties such as activators or inhibitors of growth, differentiation, etc. These molecules can be soluble in water or volatile in air. The mycelia of Dikarya can exhibit circadian growth rhythms independent of light or directly controlled by light, also allowing coordination of the entire colony. Growth is often driven by tropism (turning towards something). This includes tropism towards nutrients, optimum temperature or the phenomenon of repulsion of young hyphae frrom each other, except when developing multicellular structures. Anastomoses in fact only concern older hyphae or else very young hyphae resulting from the germination of spores, called germ tubes. The most surprising tropism described is that of several species of fungi moving towards sources of radioactive emission! This is to be put in relation with the fact that the growth of strongly melanized fungi seems to be accelerated in the presence of radiation!
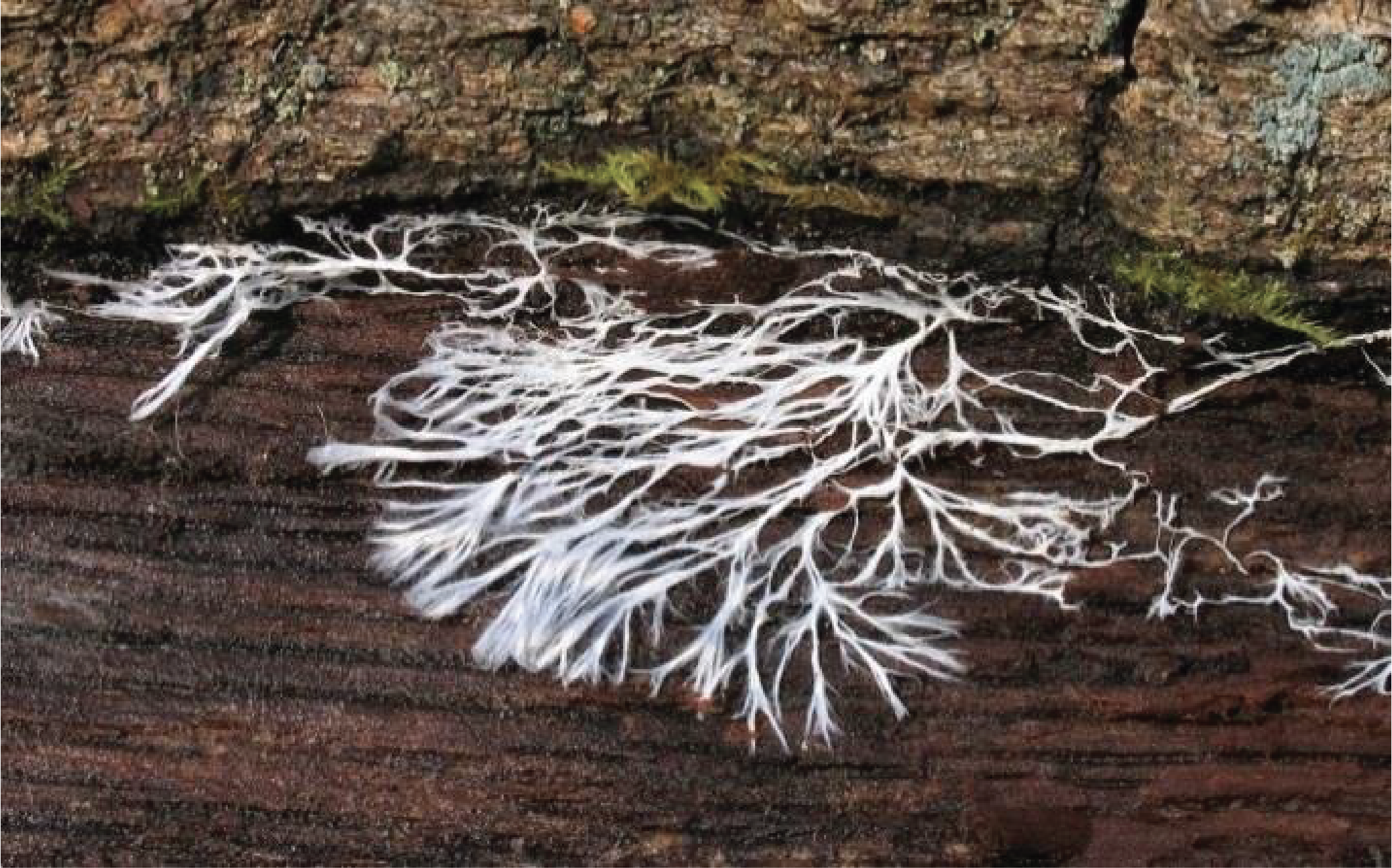
Figure 132.
Mycelial cords. These aggregated hyphal bundles allow better invasion of the substrates. When they are melanized, they are called rhizomorphs. They are rather characteristic of Agaricomycotina.The hyphae of Dikarya are also capable of recognizing self and non-self, with different mechanisms that operate when the hyphae belong to the same species or to different species. Intra-specific recognition can take two forms. In all cases, hyphae of the same species will become attractive if they are old enough. Mechanisms then prevent either the fusion of the hyphae or lead to the death of the fused cell. This phenomenon is called pre- or post-fusion vegetative incompatibility. It is under the control of genetic differences between hyphae, genetically homogeneous hyphae can fuse with each other successfully, while genetically different hyphae cannot. Interspecific recognition is related to the phenomena of innate immunity present in animals and plants and is called hyphal interference (Figure 133).
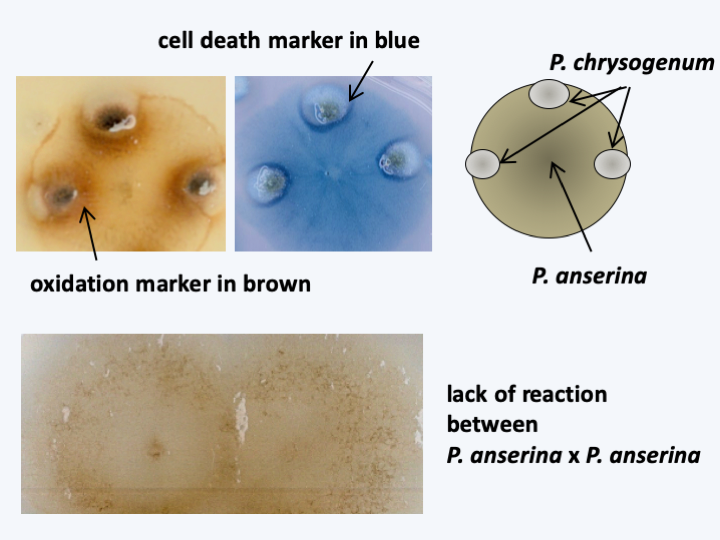
Figure 133.
Hyphal interference, a mechanism of innate immunity. Some Eumycota, like Podospora anserina, are able to differentiate self from non-self. Indeed, only in the presence of hyphae of another species, this fungi produces an oxidation which can lead to the death of the other fungus, here Penicillium chrysogenum. The hyphal interference involves a machinery common to that of plants and animals, including in particular an oxidase of NADPH and a MAP kinase pathway. Oxidation is measured by the accumulation of a brown precipitate of diaminobenzidine and cell death by that of Evans blue when the two fungi are confronted.Some species of Dikarya have an infinite capacity for vegetative growth. We cite, for example, the case of a species of Armillaria whose mycelium covers 9 km2, weighs 2,000 tonnes and whose age is estimated at 5,000 years or that of Neurospora crassa which was cultivated in vitro for two years without loss of vigor; the apex will therefore have traveled 65 m. On the contrary, some have a limited capacity to proliferate, like most coprophilic fungi, including Podospora anserina (Figure 134). There are many syndromes of degeneration of the mycelium, the causes of which are epigenetic in nature.
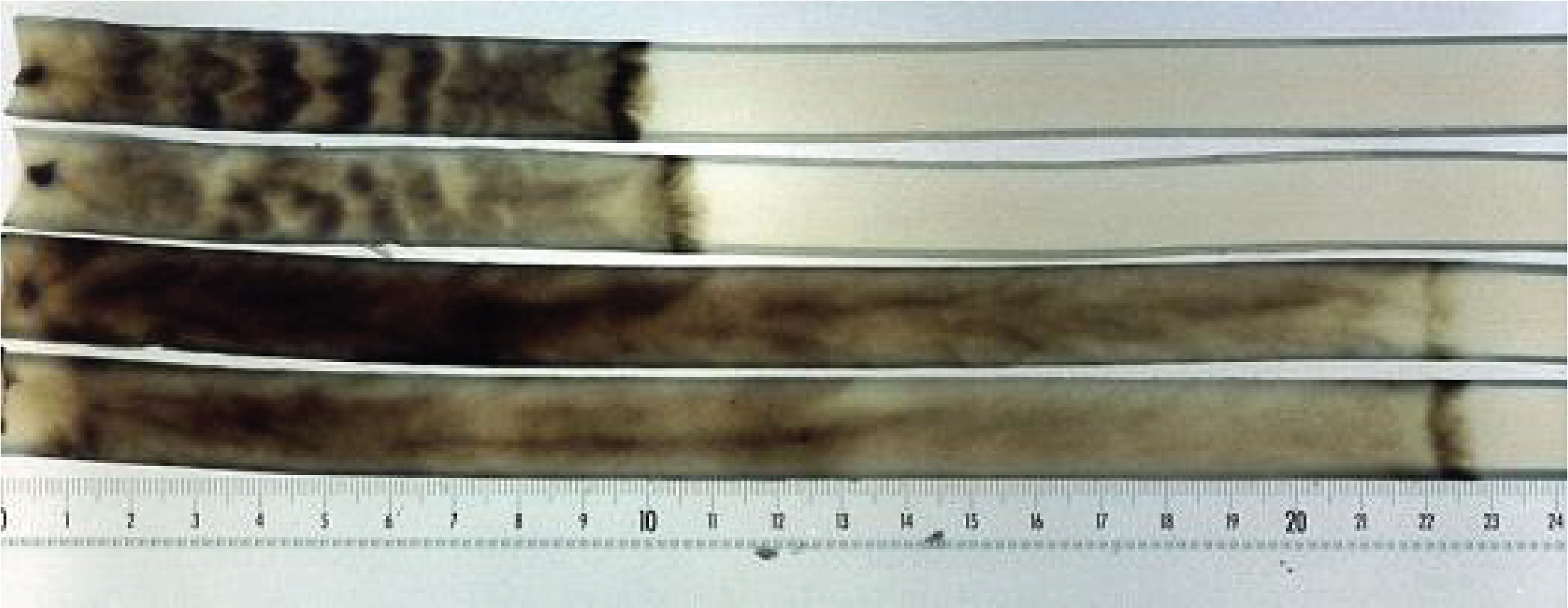
Figure 134.
Senescence of Podospora anserina. At the top, two cultures of the wild strain, at the bottom two cultures of a mutant with greater longevity. At the level of the dark bar on the right the cells are dead. The cells which die are therefore the 'youngest' because those resulting from the last divisions! In the majority of the cases analyzed, including that of Podospora anserina, senescence is correlated with a destabilization of the mitochondrial DNA which changes during divisions and ends up causing cell death by asphyxiation. The causes of this destabilization in this species and other fungi are still obscure.Many species are capable of forming multicellular vegetative thalli, such as the lichen-forming species (Figure 135). Others produce multicellular and strongly melanized structures known as sclerotia (Figure 136). When placed in the right conditions, these sclerotia will directly produce sporophores. However, it is during spore production that Eumycota differentiate the most complex multicellular structures. The production of asexual spores occurs most often on simple structures, such as the conidiophore of Aspergillus (Figure 76), or even by fragmentation of the mycelium, but in some species may involve the production of complex structures. In contrast, at the time of sexual reproduction, Eumycota very frequently differentiate very elaborate multicellular structures, where some carpophores can reach large sizes - more than 50 cm in height - and adopt complex shapes (Figure 137). The mechanisms that trigger the production of fruit bodies are still largely unknown. In Ascomycota, they involve G proteins and MAP kinase cascades. These respond to and integrate multiple signals (temperature, light, hardness of the substrate, specific deficiency, etc.) which often make it difficult in the laboratory to obtain fruiting bodies.
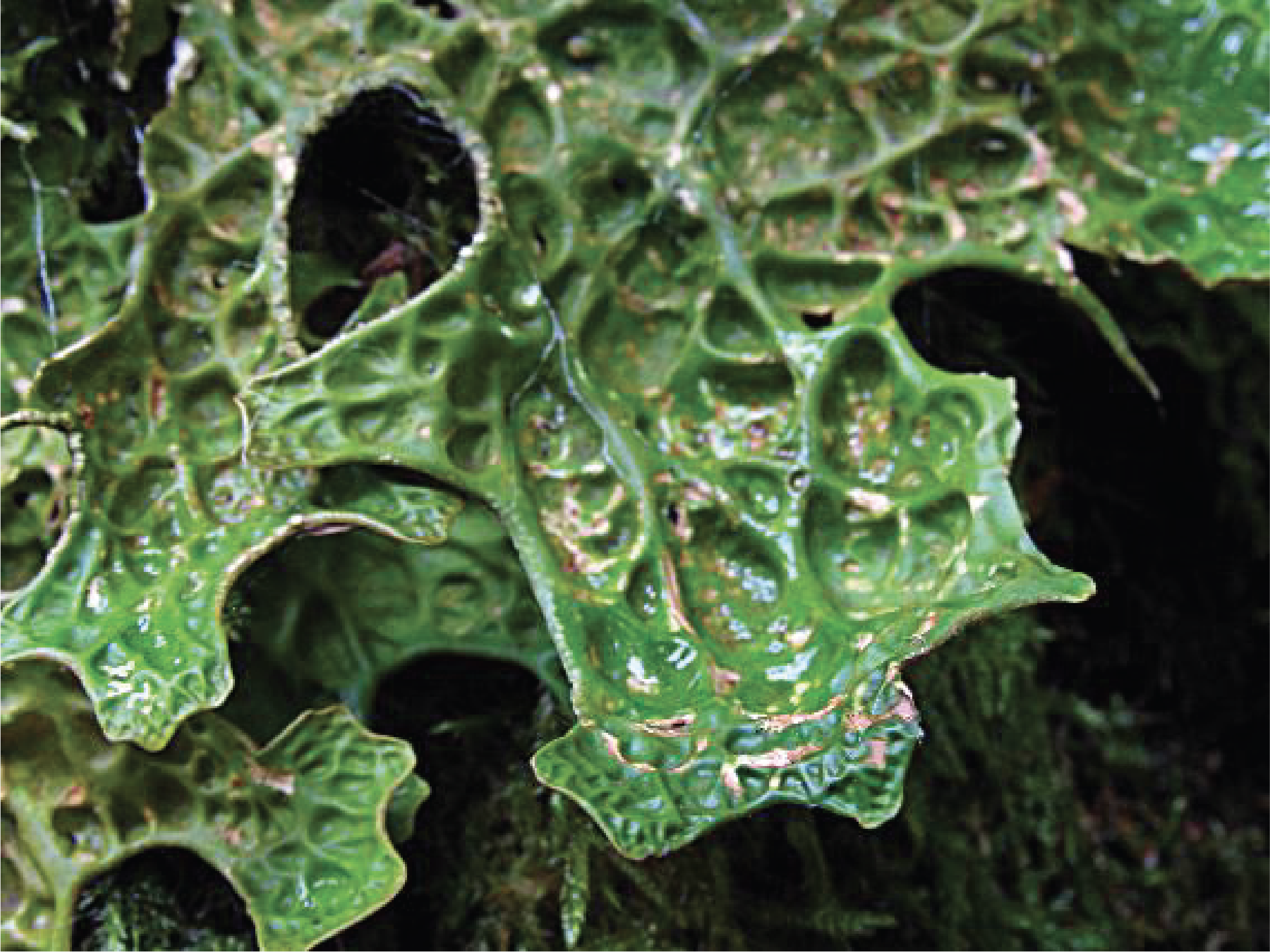
Figure 135.
Thallus of Lobaria pulmonaria. The vegetative thallus of this species, like that of most lichens, is composed of simple tissues with differentiated fungal cells.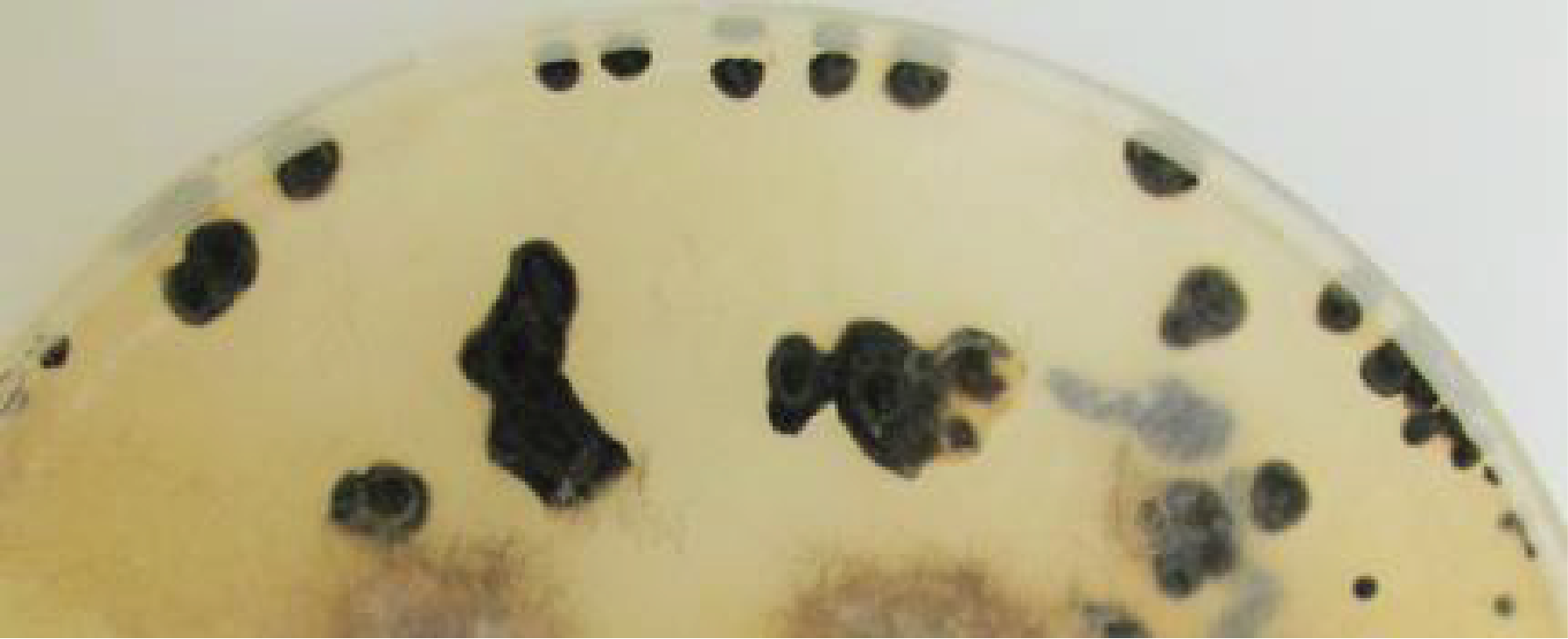
Figure 136.
Sclerotia of Botrytis cinerea. Sclerotia are compact aggregates of hyphae that will allow the fungus to get through the bad season. These sclerotia give rise to fruiting bodies at the return of the favorable season for development.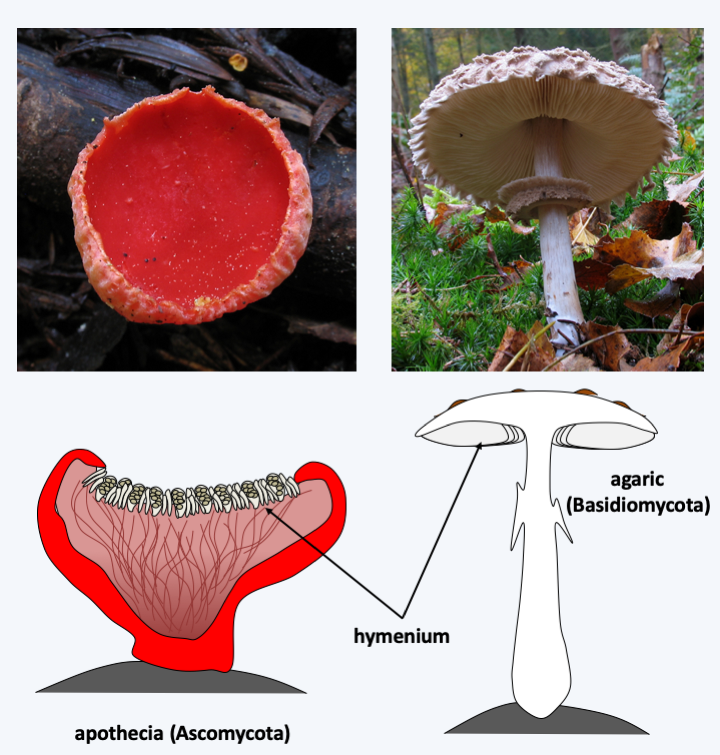
Figure 137.
Two types of complex multicellular carpophores. On the left, Sarcoscypha coccinea, an Ascomycete, produces an apothecia-type fruiting body and on the right, Macrolepiota rhacodes, a Basidiomycete, an agaric-type fruiting body. These two types of complex fruiting bodies appeared independently. In both cases, the carpophores are formed from differentiated hyphae. The hymenium is the portion of the carpophores carrying the cells that cause meiosis and originate the spores.Much remains to be discovered also of the developmental modes of fruiting bodies. Current knowledge builds largely on arrangements of hyphae with differential coloring. These sometimes show strong cellular differentiations: for example, in Neurospora crassa, a Pezizomycotina, at least 28 morphological types of hyphae can be found, most of which only in the fruiting body. It is probable that there are even more in Agaricomycotina. Most of the cells appear to be totipotent and capable of regenerating a complete individual on their own. However, there are cells that die by apoptosis during the development of some of these structures and other cells that appear to be terminally differentiated. The complex fruiting bodies are an adaptation to dispersal in the air, because like plants and animals, the Dikarya have conquered the aerial environment. Their development is therefore partly driven by the efficiency of their dispersion of spores in the air.
Due to their integrated mycelia and multicellular fruiting bodies, Dikarya are, like animals and plants, capable of complex behaviors. In fact, the mycelial thallus of Dikarya is not a single colony. It is a network of interconnected cells that can exchange nutrients and information. The thallus is able to sense its surroundings and set up appropriate responses. The data therefore indicate that a mycelial thallus is more like an individual. For example, when cultivated in certain media, Podospora anserina shows a non-random distribution of its fruiting bodies contrary to what is expected if the thallus were homogeneous (Figure 138).
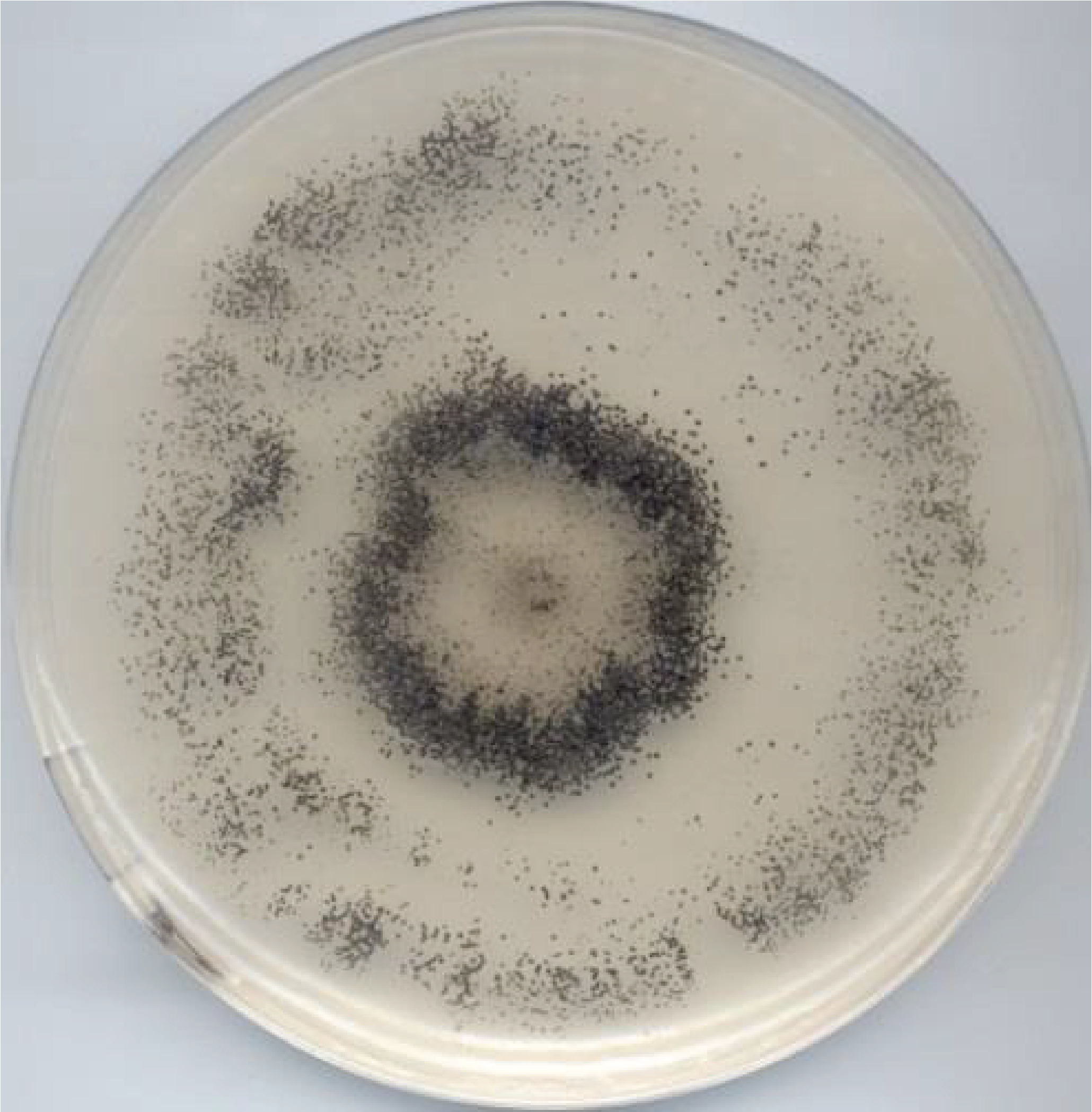
Figure 138.
Distribution of fruiting bodies on a thallus of Podospora anserina. The fruiting bodies (the little black dots) are distributed mainly in a circle which is reminiscent of witch circles.Beside complex multicellularity, metabolic innovations probably contributed to the evolutionary success of Dikarya. In particular, the ability of some species to degrade lignin has enabled them to access carbon sources that other saprotrophs cannot use. This ability has involved the evolution of an enzymatic machinery capable of attacking polymers in a non-specific way. The emergence of Dikarya effective in degrading lignocellulose dates from the end of the Carboniferous period, the period when plant matter accumulated in the sediments to end up as the carbon we use as fossil fuel. It is therefore very likely that the appearance of the efficient Dikarya degradation system has since prevented the accumulation of fossilized coal except in a few ecosystems, where these fungi perform poorly, such as in peatlands. The Dikarya also quickly associated with photosynthetic organisms, be they cyanobacteria or algae, to give rise to lichens, or to plants that they colonize as pathogens or as mutualists. In fact, they form, for example, ectomycorrhizae with the majority of trees in boreal forests but also with some trees in tropical forests. The associations they initiate do not stop with plants, since some live as commensals, mutualists or parasites of animals, protozoa or other fungi. Changes in trophic modes are very frequent within different lineages and the same types of associations are formed in recurrent ways. There are for example Basidiomycota lichens, although the majority of them are formed by Ascomycota, and the proportions are reversed for ectomycorrhizal fungi, because most of them belong to the Agaricomycotina subphylum of Basidiomycota. The case of nitrate assimilation in Trichoderma is interesting from this point of view. This is because the use of nitrate requires a nitrate transporter, a nitrate reductase which reduces nitrate (NO3) to nitrite (NO2), and a nitrite reductase which reduces nitrite to ammonium (NH4+). These three genes are often encoded as a “cluster” and appear to have been transferred to Dikarya via horizontal transfer, possibly from an oomycete. Indeed, these three genes are lacking in non-Dikarya, and have been lost in some Dikarya yeasts. The use of nitrate as a source of nitrogen has probably enabled the Dikarya to eat better in the nitrogen-poor environments that constitute their biotopes. Further proof of the importance of this cluster is its absence in Trichoderma, which are exclusively endophytic and parasitic fungi, thereby having access to nitrogen via their plant or fungal host. Trichoderma reesei is an exception because it has a cluster that strongly resembles that of Basidiomycota, which are often parasitized by Trichoderma. Trichoderma reesei is the only saprotrophic species of the genus. It is thus probable that it was able to free itself from its host in part through the acquisition of the nitrate assimilation cluster.
Due to their ecological importance, their metabolic capacities and their ability to degrade, Dikarya have a significant impact on human society. Some are used for consumption, like button mushrooms, truffles, morels, chanterelles, porcini mushrooms… The markets for these mushrooms can be very important: The white truffle of Italy - Tuber magnatum - can reach the price of 30,000 € / kg! Black Périgord truffle - Tuber melanosporum - 2,800 €/kg! The prices noted in Paris in December 2006 are shown inFigure 139. It is therefore not surprising that murders can be committed in the name of these fungi! The porcini - Boletus edulis - can be worth up to € 200/kg and is usually traded at € 20 / kg. Annually, around 2,000,000 tonnes of button mushrooms are consumed, which sell for € 2/kg, 1,500,000 tonnes of Shiitake at € 10/kg and 900,000 tonnes of oyster mushrooms at € 5/kg. About 1,000,000 tonnes of mushrooms belonging to other species are traded. This estimate does not take into account an indefinite quantity of mushrooms which are directly consumed after having been collected during Sunday walks …
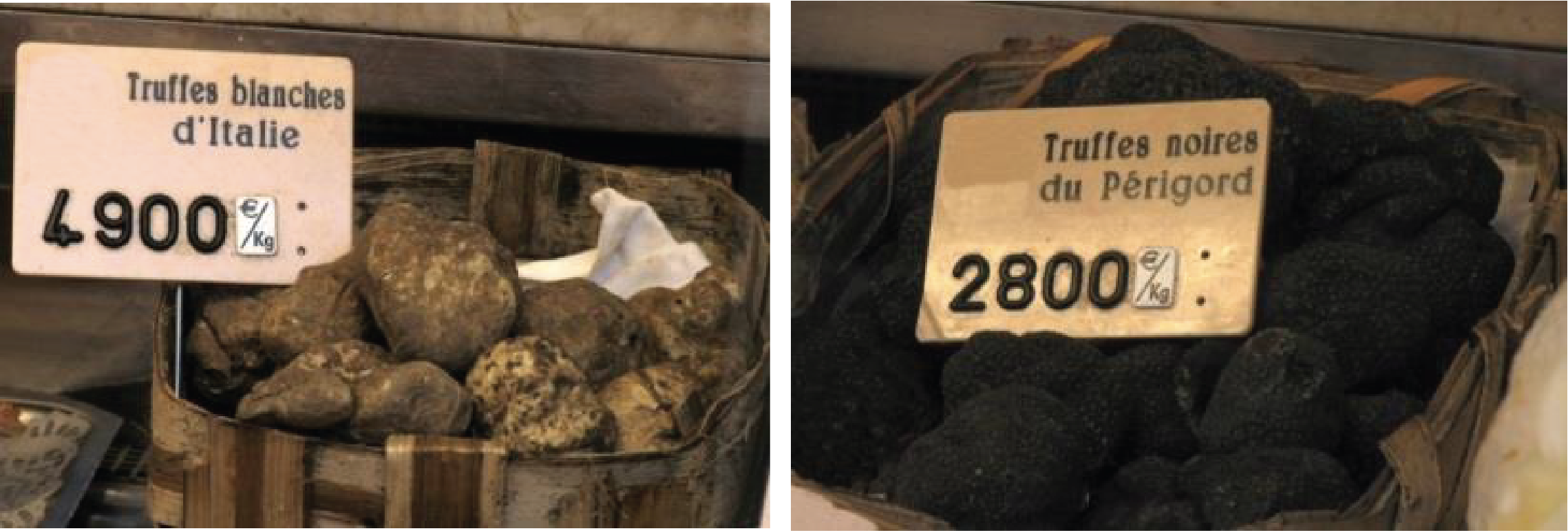
Figure 139.
Carpophores of truffles can fetch very high prices. Prices are in euros per kilo.Some species are used for food processing. This is particularly the case of the yeast Saccharomyces cerevisiae used for the manufacture of bread and alcoholic beverages such as wine, beer or cider. Many other species are involved in the ripening of cheeses, such as Camembert or Roquefort, or the production of fermented products such as chocolate or soy sauce.
The Dikarya make many substances with biological effects. They are most often derived from secondary metabolism, but some such as plectasin, a peptide secreted by an Ascomycota and possessing a strong antibacterial activity like the peptides produced by the innate defense system of animals, is produced via cytosolic translation. Often these products are very toxic and can be fatal in very low doses. They are then subject to control if they enter the food chain. Some are used by humans as medicines. In the 1990s, among the 20 most prescribed drugs, six, including of course penicillin but also cyclosporine preventing transplant rejection and lovastatin with anti-cholestreolemic activity, had a fungal origin. Dikarya also produce many enzymes whose properties are of interest to manufacturers. In particular, they are the only ones to produce enzymes which can be used in the paper industry, such as those which degrade lignin and which therefore “whiten” the paper properly. Great hopes are also placed on these enzymes for future technologies, with the possibility, for example, of using them to manufacture biodegradable batteries.
The effects of Dikarya on human societies are not only beneficial. It is among this group of organisms that the vast majority of fungi responsible for yeast infections, the human diseases caused by fungi, are found. Collectively, fungi currently kill more people than malaria! Animals, especially those we breed, are not left out. However, due to their temperature optimum below 37°C, fungal impact on warm-blooded animals is less than on cold-blooded ones. The plants we grow are not spared either, as some of the major plant pathogens are Dikarya. About 80% of plant diseases are caused by fungi of this group. Stored crops are ideal foods for certain species, which destroy a large amount; look in your fridge! The same goes for many wooden objects such as frames, old paper or sculptures. An English ship in the 19th century even had to be destroyed before its launch because it was eaten by mushroom-forming fungi before the end of its construction! Because of their diversity and their importance, we will look into the phyla of Dikarya, Ascomycota and Basidiomycota, in more detail.
Back to chapter index