Mitochondria
Back to main indexThe origin of mitochondria no longer seems to present a problem. Indeed, it is clear to the entire scientific community that it derives from the endosymbiosis of a bacterium of the α-proteobacteria class in a precursor cell. The central argument in favor of this hypothesis is that the mitochondria has its own genetic system, that is, it functions like a very simplified bacterium. In particular, the mitochondrial DNA sequences of protozoa of the Jakobida class (branch of Excavata), such as Reclinomonas americana (Figure 24) or Andalucia godoyi, provide very interesting information. These protists have mitochondrial genomes encoding around 100 genes, which is the highest observed number in any mitochondrial genomes. In fact, all the mitochondrial genes present in other eukaryotes are found in the mitochondrial genomes of Jakobida. These contain, in addition to what is usually encoded by the mitochondrial genome such as ribosomal RNAs and some subunits of respiratory complexes, a gene for 5S rRNA, a gene for the RNA component of RNAse P which is involved in the maturation of tRNAs, genes encoding ribosomal proteins, respiratory chain electron transport subunits, transporters and a cytochrome c1 maturation protein. Even more interestingly, it also contains the secY export system bacterial proteins and 4 genes which code for a bacterial-like RNA polymerase. These latter genes are also very interesting because until the description of these genomes, the mitochondrial RNA polymerases were encoded by the nucleus and had a viral RNA polymerase structure. Jakobida mitochondrial genomes therefore resemble bacterial mini genomes, thus confirming the endosymbiotic origin of the mitochondria. Comparison of mitochondrial DNA sequences, or genes encoded by the nucleus but whose products function in the mitochondria, in many organisms show that these genes most often resemble those of α-proteobacteria (Figure 25). The extant bacterium having the genome closest to that of the mitochondria is a free marine rickettsia, Candidatus Pelagibacter communis (the species has not yet been formally described according to the bacteriological code). However, many Rickettsiales are intracellular pathogens (Rickettsia sp.) or endosymbionts (Wolbachia sp.). The tree obtained shows a single root with strong statistical support, indicating a single origin of the mitochondria. However, when the genes are analyzed separately, some genes seem to come from other α-proteobacteria such as Rhizobiales. Note that the origin of the mitochondrial double membrane is not clear (Figure 23), as there could have been three membranes at some point at the origin of mitochondria. Two hypotheses can be envisioned. First, both membranes result from the double membrane of the proteobacterium, either by entrance of the bacterium directly into the cytosol without an endocytosis vacuoule, or through escape of the bacterium from the lysosome, as some bacteria currently are known to do. Second, the outer membrane of the mitochondria results from the endocytosis vacuole, and either the outer or inner membrane of the proteobacterium would have instead regressed.
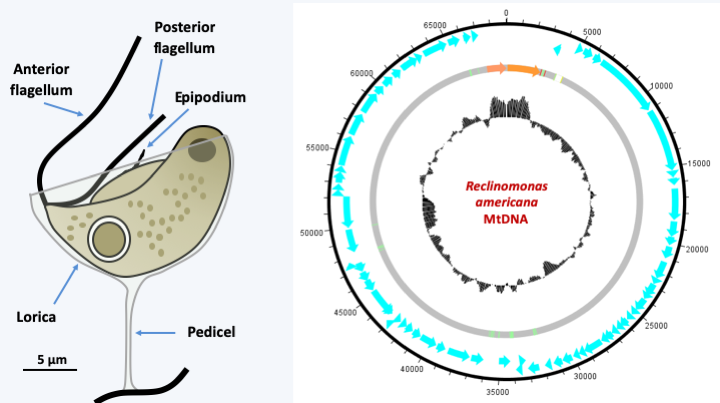
Figure 24.
Reclinomonas americana and its mitochondrial genome. R. americana is a cosmopolitan freshwater protozoan. It is most often embedded in an organic theca which attaches it to the substrate via a pedicel. The epipodium is a structure supported by microtubules but is not a third flagellum. Its mitochondrial genome is one of the largest known; in blue, the genes encoding proteins, in orange, the ribosomal RNA genes and in green the tRNA genes; the central circle represents the percentage in base GC.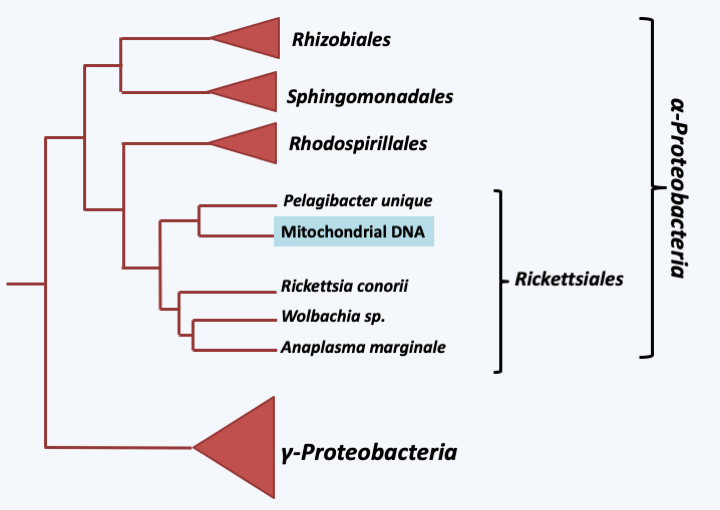
Figure 25.
Phylogenetic tree of mitochondrial and bacterial genomes. Mitochondrial DNAs have a common origin within Rickettsiales.All current data therefore indicate that a single endosymbiosis event is at the origin of the mitochondria, suggesting that this event gave the ancestral eukaryote a very important selective advantage, allowing it to quickly replace all its competitors. What can be the benefits of mitochondria? They diverge depending on the theory adopted for the appearance of the eukaryotic cell. In syntrophic theories, the postulated benefit might initially be the efficient delivery of H2 to the methanogenic partner. Then when switching to aerobic respiration, the mitochondria could have provided an important source of energy to the cell. Another theory suggests that the presence of mitochondria would have allowed the evolution of the eukaryotic cell, by generating a reserve of ATP which could have been used for the complexification of the eukaryotic genome, unlike prokaryotes which would constantly use ATP which they produce to replicate their DNA and ensure the synthesis of other macromolecules. Prokaryotes would therefore be under selective pressure to move towards compact and efficient genomes, unlike eukaryotes. This hypothesis was tested by measuring the growth of bacteria that contain multi-copy plasmids of different sizes, which mimics the increase of the genome size, and it turns out that there is indeed an inverse correlation between the size of the plasmid, the frequency with which it is lost and the number of bacteria obtained after growth in a medium with a constant amount of nutrients. However, this hypothesis assumes that the mitochondria provided ATP to its host from the start, which for the proto-mitochondria should have been strongly counter-selective. The theory that I favor as discussed in the previous chapter suggests that initially the mitochondria could have detoxified oxygen and allowed the proliferation of the initially obligate anaerobic proto-eukaryote. This mechanism would have been particularly important at the time of oxygen release after the invention of oxygenic photosynthesis by cyanobacteria, which would place the origin of mitochondria and therefore eukaryotes to around 2 billion years ago. The supply of ATP would under this hypothesis be a subsequent positive effect because the final establishment of mitochondria as an energy supplier required an important invention: the ADP/ATP translocator which allows the release of ATP and entry of ADP into the mitochondria (Figure 26). This invention appears to originate from the eukaryotic host because sequence comparisons show that the ATP / ADP exchanger is close to broader spectrum transporters found in eukaryotes. This thus allowed the host cell to enslave the symbiotic bacteria. Note that in addition to respiration, the acquisition of mitochondria may have been accompanied by the acquisition of other functions. Indeed, the mitochondria is the site of many metabolic reactions. It ensures the maturation of the iron-sulfur centers necessary for the functioning of several enzymes essential to the cell and involved in various cellular processes including the modification of transfer RNAs, the urea cycle, the production of energy in the mitochondria or the biosynthesis of heme, cofactors and amino acids. Surprisingly, the respiratory activity of the mitochondria is dispensable while other mitochondrial functions are essential. For example in the yeast Saccharomyces cerevisiae, a facultative aerobe, mitochondria are required, although this yeast can lose its mitochondrial DNA, resulting in an absence of respiration. Another contribution of the mitochondria would be the establishment of type 1 apoptosis. In fact, this type of apoptosis, present in very many eukaryotes, is triggered by a modification of the permeability of the outer mitochondrial membrane. This could be a relic of a defense system put in place by the ancestor of mitochondria (see previous chapter). Its control by the eukaryotic cell allowing it once again to make its development or defense mechanisms more complex.
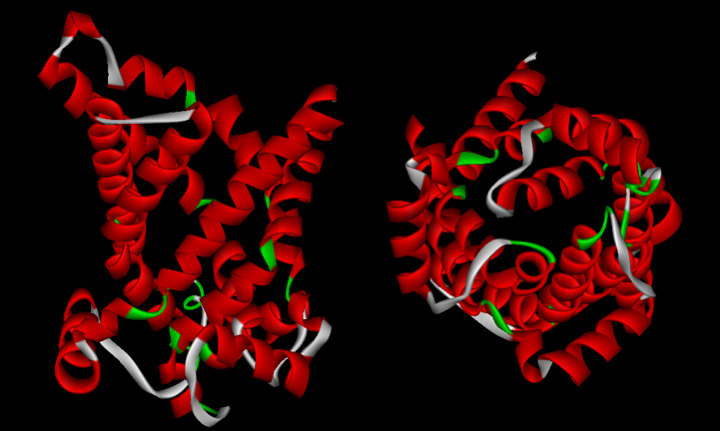
Figure 26.
The ATP / ADP translocator of yeast mitochondria. This protein forms a pore in the mitochondrial inner membrane and allows the entry of ADP and the exit of ATP into the mitochondria. It belongs to a family of transporters present mainly in eukaryotes, but some members of which are found in eubacteria.After the initial endosymbiosis, the proto-mitochondria underwent a simplifying (or even reducing) evolution which was accompanied by a loss of its genes or their transfer to the nucleus. According to the theory of phagotrophic origin, it is this process that is primarily responsible for the presence of functional genes of mitochondrial origin in eukaryotic nuclear genomes. This process of reductive evolution usually occurs in the case of intracellular parasites, such as Rickettsia prowazekii. Indeed, the comparison of the genomes of the eubacterium Escherichia coli, of Rickettsia prowazekii and of the mitochondria shows that the latter two genomes have lost genes compared to that of Escherichia coli. However, the process of loss is different between Rickettsia prowazekii and mitochondria. Likewise, the losses occurred differently between the different eukaryotic lineages as shown inFigure 27, which represents the set of genes found in the mitochondria in different eukaryotes. Note that the last genes to go are ribosomal RNAs, cytochrome B and two cytochrome oxidase subunits. It appears that cytochrome B and cytochrome oxidase subunits 1 and 3 can only be properly inserted into the inner membrane of the mitochondria, the site of oxidative phosphorylation, by co-translation. They must therefore be translated inside the mitochondrial matrix, hence the need to keep the two ribosomal RNAs, the genes for cytochrome B and subunits 1 and 3 cytochrome oxidase in the mitochondrial genome. These subunits cannot pass through the membrane because they are too large. In today’s mitochondria, the majority of proteins (99% of the approximately 800 proteins functioning in the mitochondria) are encoded in the nucleus; the mitochondria has long ceased to be a bacterial symbiont, and has instead become a fully integrated functioning organelle.
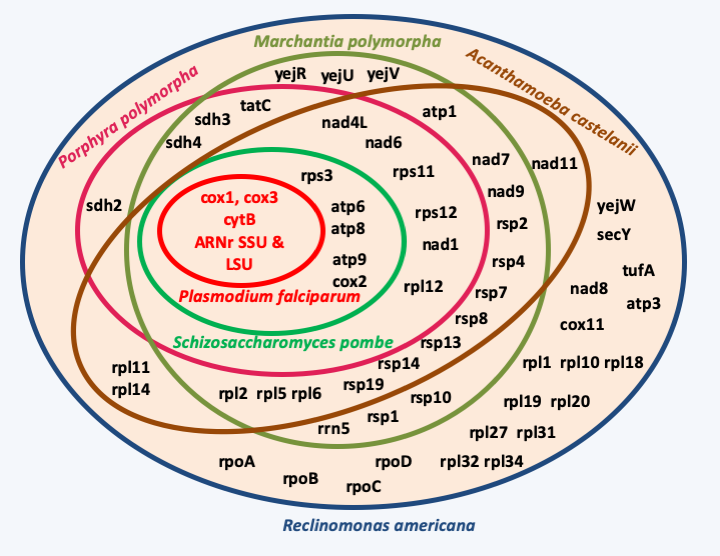
Figure 27.
The gene content of mitochondria is variable depending on the phylogenetic groups.From a mechanistic point of view, the fine modalities of transfer of mitochondrial genes to the nucleus is unknown. This process can be detected in the yeast Saccharomyces cerevisiae with the experiment described in Box 4. This phenomenon is frequent in this yeast, which accounts for the unidirectional transfer from the mitochondria to the nucleus. However, recent analyzes show that in Saccharomyces cerevisiae, a good half of the proteins located in the mitochondria do not have a bacterial homolog. This protein supply to the mitochondrial compartment would come in part from eukaryotic host such as the ATP/ADP translocator or the ancillary actors of the TOM and TIM systems which allow the transport of proteins through the external and internal membranes. The hearts of these two systems would come from bacterial transporters having changed direction. The other part would come in part from gene replacements by viral genes, such as RNA polymerase in the majority of eukaryotes, or genes resulting from horizontal transfers from other bacteria. In fact, 40% of the proteins functioning in the mitochondria appear to originate from various eubacteria and archaea.
Box 4. Transfer of genetic material between nucleus and mitochondria
The transfer of DNA from the mitochondria to the nucleus can be easily demonstrated using the yeast Saccharomyces cerevisiae. To do this this, yeasts incapable of synthesizing uracil and unable to perform respiration, by carrying the nuclear mutation URA3 and the mitochondrial mutation mit-, are transformed with DNA containing the wildtype alleles of the URA3+ and mit+ genes, under conditions where the transformed DNA enters the mitochondria. Transformants capable of respiration are selected on medium containing glycerol as the sole carbon source. Since glycerol is not metabolized via glycolysis, yeast cells that cannot breathe cannot grow with this carbon source. These [mit +] transformants, although containing the URA3+ allele in the mitochondria, do not grow on uracil-free media because the different genetic code of the mitochondria prevents the correct expression of this nuclear gene. After plating on a medium without uracil, a few colonies appear. Most correspond to the transfer of the URA3+ allele to the nuclear genome. This experiment shows that transfers are frequent, because colonies appear with a high frequency of the order of 2×10-5 per generation. This level is decreased in mutants altered for the vacuole, suggesting that DNA escapes to the nucleus when mitochondria are degraded, such as when respiratory requirements decrease.
Subsequently, for it to be expressed in the nucleus, it is necessary that the transferred coding sequence captures an addressing sequence to the mitochondria and is subjected to appropriate expression. Likewise, at present, certain codons must be corrected, because they are read differently in the nucleus and in the mitochondria. Indeed, the reduction of the mitochondrial genome was accompanied by modifications of the mitochondrial genetic code, making it different from the standard genetic code (see below and Table 2). It therefore seems that at present, mitochondria-nucleus transfers are very difficult. The simplification of the coding capacity of mitochondrial genomes is often accompanied by the appearance of non-coding sequences, in particular the massive arrival of intergenic sequences of unknown function, some of which clearly derive from the integration of plasmids, or of introns (Figure 28). These introns resemble introns found in bacteria and mainly belong to two distinct families. One of the two families produces a linear intron as a product of the splicing reaction (group I) and the other a branched molecule or lariat such as nuclear introns (group II). Most contain one or two coding sequences that are often involved in splicing the intron that contains them. Group I introns encode proteins with endonuclease activity and group II introns have reverse transcriptase activity. These activities allow mobility of introns which are therefore also transposons. The size of mitochondrial genomes is therefore not always directly related to their coding capacity (Figure 29). In addition, changes in genome structure and coding or expression mechanisms can result in very different ways of functioning from other genomes (Box 5). Most mitochondrial genomes are circular but in some cases they can be linear (Figure 29) or in the form of several chromosomes (Box 5).
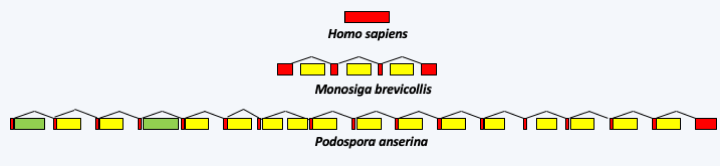
Figure 28.
The structure of the gene encoding cytochrome oxidase subunit 1 (cox1) in 3 organisms. In humans, the coding sequence does not contain an intron, while in the choanoflagellate Monosiga brevicollis, it contains three group I introns. In the Ascomycete fungus Podospora anserina, it contains two group II introns (in green) and fifteen group I introns (in yellow).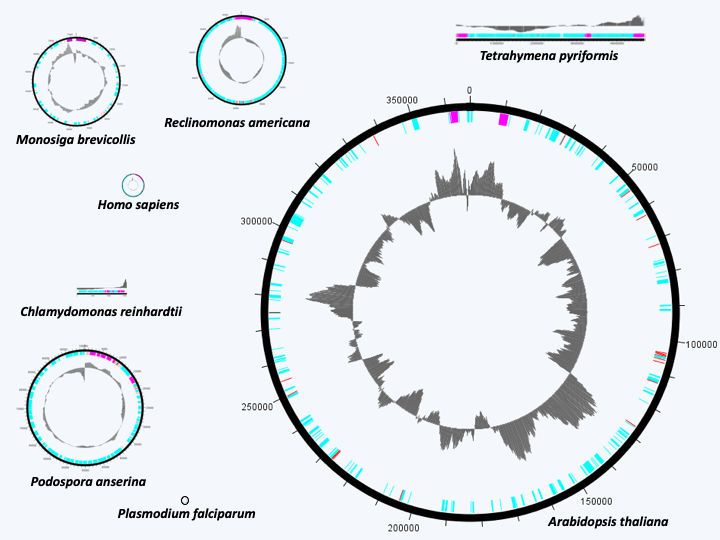
Figure 29.
The size of mitochondrial genomes is highly variable and is not always related to their coding capacities. The genome of Reclinomonas americana has the most genes, but is much smaller than that of Arabidopsis thaliana. The smallest known mitochondrial genomes are those of Plasmodium which are only a few kilobases. They code for 5 genes. In the center, GC%.Box 5. Strange mitochondrial genomes
Mitochondrial genomes can take on very strange structures and patterns of expression. In Kinetoplastea, the mitochondrial genome, called kinetoplast, is made up of two different types of molecules: the 'maxicircle', present in about ten copies, and about 200 different 'minicircles', collectively present in several thousand copies. The molecules are entangled with each other and do not exhibit superturns, which is unique in the living world. The maxicircle codes pre-mRNA that do not have a complete coding phase. Following correction to the pre-mRNA (editing), made possible by the use of guide RNAs encoded by the minicircles, the final mRNAs can be translated. In some species, up to 80% of the nucleotides in pre-mRNA can be changed!
There is no rule for the structure of these genomes because for each group they have undergone unique evolution. These important changes during evolution are probably related to the fact that there are generally tens, or even thousands, of mitochondrial DNA molecules per cell and that therefore for each of these molecules the selection pressure is relaxed, allowing their rapid evolution. The presence of introns also having a transposon behavior in these genomes allows both their fragmentation and their rearrangement while maintaining the coding capacity by trans-splicing or editing. The decrease in the coding capacity of mitochondrial genomes has also allowed frequent modifications of the genetic code; 13 different mitochondrial genetic codes are currently known, where up to 6 codons have been reassigned. Table 2 gives that of the mitochondria of Saccharomyces cerevisiae. Modifications are rare for the nuclear code. In some Saccharomycotina yeasts CUG encodes serine rather than leucine and in some Ciliophora stop codons encode either glutamine or cysteine depending on the phylogenetic group.
The extreme specification of the mitochondria has led to very specific modalities of functioning in certain organisms. Classical mitochondrial energy physiology is summarized inFigure 30. In the majority of cells, the preferred carbon source is glucose. In others, fatty acids are preferred. Glucose is first converted to pyruvate via glycolysis, which makes it possible to produce two molecules of ATP per molecule of glucose. Glycolysis also produces two reducing equivalents (NADH, H+) per molecule of glucose. Since glycolysis does not require oxygen, it occurs in all cells, whether they are aerobic or anaerobic. In the presence of oxygen, the mitochondria can take over by decarboxylating pyruvate to acetyl-CoA which then enters the Krebs cycle. Ultimately, the mitochondria break down pyruvate into carbon dioxide and water. The breakdown of fatty acids also results in acetyl-CoA upstream of the Krebs cycle. The coupling between the degradation of acetyl-CoA and the respiratory chain allows the production of about thirty ATP molecules per initial glucose molecule. In anaerobic condition, the mitochondria can no longer function normally. Cellular metabolism therefore switches to the fermentation pathway, which in eukaryotes are either lactic, in mammals for example, or alcoholic, in the yeasts Saccharomyces cerevisiae or Schizosaccharomyces pombe for example. This route does not produce ATP but uses the two reducing equivalents. In some species, mitochondria exhibit functional plasticity (Table 3) and may under certain conditions where oxygen is lacking or during certain stages of development use alternative donors or electron acceptors. However, in the presence of oxygen at other stages of development, these organisms are perfectly capable of breathing with oxygen. Mitochondrial plasticity is brought about by changes in the proteins of respiratory complexes and the presence of proteins capable of accepting electrons such as cytochrome P450 or alternative oxidase. This last protein is found in many eukaryotes, where it seems to act at the time of respiratory dysfunctions. Its expression helps bypass the flow of electrons, thereby reducing the production of toxic oxygen compounds, such as superoxides and peroxides.
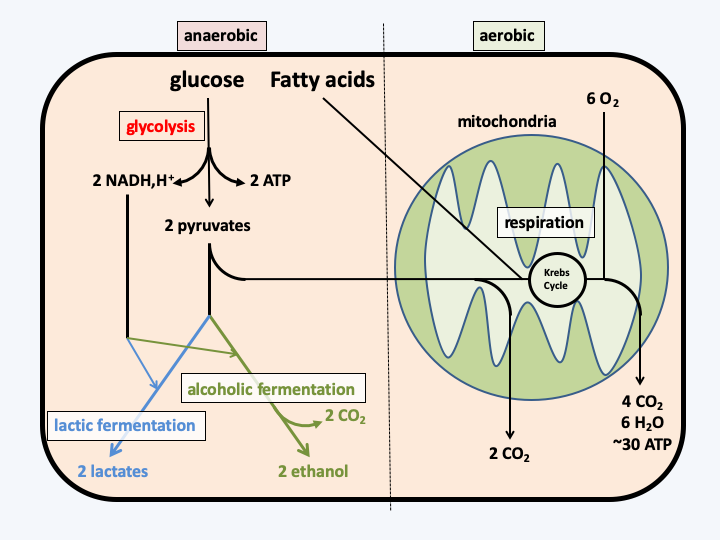
Figure 30.
Energy metabolism typical of the eukaryotic cell.As seen in the chapter dealing with the appearance of the eukaryotic cell, in groups living in strict anaerobic conditions, the mitochondria may have undergone more significant changes. It was thus able to specialize in the production of hydrogen, thus becoming a hydrogenosome or else completely losing the energy production activity and becoming a mitosome, where only the assembly of iron/sulfur complexes seems to occur. We even know of an organism (Blastocystis hominis), in which the mitochondria is also a hydrogenosome and another (Psalteriomonas lanterna) in which the hydrogenosome seems to be a more “mature” form of mitochondria! Multiple data confirms that all of these organelles are related:
- The reactions carried out by hydrogenosomes resemble those carried out by mitochondria when they are momentarily deprived of oxygen. Likewise, if O2 is present, the hydrogenosomes reduce it, probably to H2O2 and O2-; this results in the rapid inactivation of the organelle. Like mitochondria, there is a pH gradient between the inside and the outside, the inside being alkaline, and a difference in transmembrane potential. This gradient could be used for the transport of metabolites.
- In species possessing hydrogenosomes or mitosomes, the typically mitochondrial proteins encoded by the nucleus, such as the cnp60 chaperone involved in the correct folding of the proteins imported into the mitochondria, were detected by immunodetection in the organelles. In addition, it seems that the transport modalities are homologous between the mitochondria and the hydrogenosome of Trichomonas vaginalis (the core proteins of the transport system are identical). As the transport system is complex, it is unlikely that it has appeared twice independently.
- In Nyctotherus ovalis, a Ciliophora, a genome has been discovered in the hydrogenosome. This encodes at least ribosomal RNA of the small subunit. Analysis of this sequence shows that it closely resembles the ribosomal RNAs of the small subunit of the mitochondrial ribosome of other Ciliophora species, confirming the relatedness between hydrogenosomes and mitochondria. The same goes for Blastocystis hominis, the sequence of the genome present in the organism shows that it is clearly a mitochondria capable of producing hydrogen.
The modes of operation of the hydrogenosomes are not identical between the different anaerobic groups (Figure 31), indicating independent origins for the hydrogenosomes of the different phylogenetic groups. In all cases, the energy production associated with the hydrogenosomes appears to be low. For example in Trichomonas, there is no phosphorylation linked to electron transport and therefore one mole of ATP is produced from one mole of pyruvate. The percentage of pyruvate treated by the organelle varies from 50% to a few percent depending on the species. This implies that a maximum of one additional mole of ATP per mole of hexose is produced, or a maximum gain of 30%. In vitro in Piromyces sp. E2, only 65% of the carbohydrates are degraded by the hydrogenosome into acetate and formate, the remaining 35% are degraded in the cytoplasm by various fermentation routes. In this fungus, hydrogenase is mainly involved in recycling the electron acceptors NADH and NADPH and therefore appears to have a minor role.
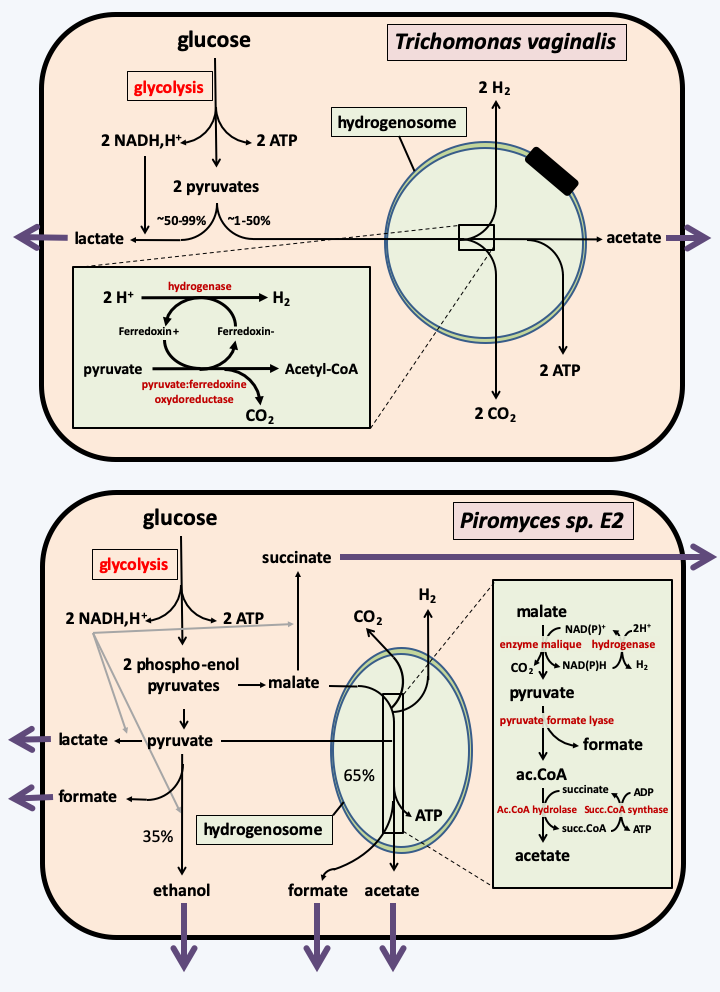
Figure 31.
Two types of hydrogenosomes. The hydrogenosome of the protozoan parasite Trichomonas vaginalis uses pyruvate, a pyruvate: ferredoxin oxidoreductase, a ferredoxin and a hydrogenase to produce hydrogen. The hydrogenosome of the fungus Piromyces sp. E2 uses malate, the malic enzyme and a hydrogenase.The ultimate regression of the mitochondria is the mitosome that has lost all energy-producing activity. This evolution has occurred several times independently, in Amoebozoa, Metamonada or Rhizaria. As in the case of hydrogenosomes, a set of facts leads to arriving at this conclusion, including the localization inside the mitosome of typically mitochondrial proteins and the conservation of proteins involved in the mitochondrial import and which participate in the import in the mitosome. In Giardia intestinalis, only the assembly of iron/sulfur centers seems to occur in the mitosome. Energy appears to be produced primarily through glycolysis and alcoholic fermentation. However, this organism has some Krebs cycle enzymes as well as a pyruvate: ferredoxin oxidoreducase and a hydrogenase. These enzymes are located in the cytosol and allow the former to metabolize citrate and malate which are involved in various interconnected metabolic pathways, and for the latter to produce hydrogen. In Entamoeba histolytica, the mitosome appears to have lost its role in the assembly of iron/sulfur centers. A possible explanation is because horizontal transfer replaced the mitochondrial assembly system (ISC system) with a different system from a eubacterium (NIF system) in this parasite. On the other hand, the mitosome seems to have gained the metabolic pathway allowing the assimilation of sulphate, again via a horizontal transfer probably originating from a eubacterium! The latter role appears to be the main function of the mitosome in Entamoeba histolytica.
All the modifications of the genome and of the mode of functioning of mitochondria, associated with the cytological observation of the characteristics of the mitochondrial morphology, make it possible to trace the evolution of the mitochondria to the evolution of eukaryotes. In the past, three major groups of eukaryotes were recognized based on the morphology of the inner membrane ridges: those that are discoidal, those that are tubular, and those that are flattened (Figure 32). Since then, molecular phylogenies have shown that this trait does not fully reflect evolution. Phylogenies based on proteins that function in the mitochondria, but mostly encoded by the nucleus, show congruence with those established from other characters, especially those from other highly conserved genes (Figure 33). These phylogenies show that eukaryotes divide into three major evolutionary lineages: Excavata, Amorphea (also called Unikonta) and Diaphoretickes. However, the oldest branches are not always supported by high probabilities. We must therefore take the order of branching of the three groups, even the order of the branches within each of the groups, with care. In the phylogeny ofFigure 33, the root of eukaryotes is placed between Bikonta and Amorphea. Other phylogenies that are not based on mitochondrial proteins tend instead to be rooted between the Excavata and other eukaryotes. Likewise, in this phylogeny, the Hacrobia are grouped together with the “SAR” (Stramenopila + Rhizaria + Alveolata). Other data indicate a closer association with the Archaeplastida, or even a polyphyletic origin of this group!
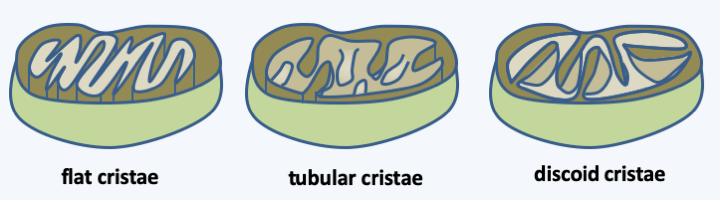
Figure 32.
The three morphologies of mitochondrial cristae. In the past, the morphology of mitochondrial cristae was used as the basis for a comprehensive classification of eukaryotes. Molecular phylogenies have shown that this classification does not reflect true phylogeny.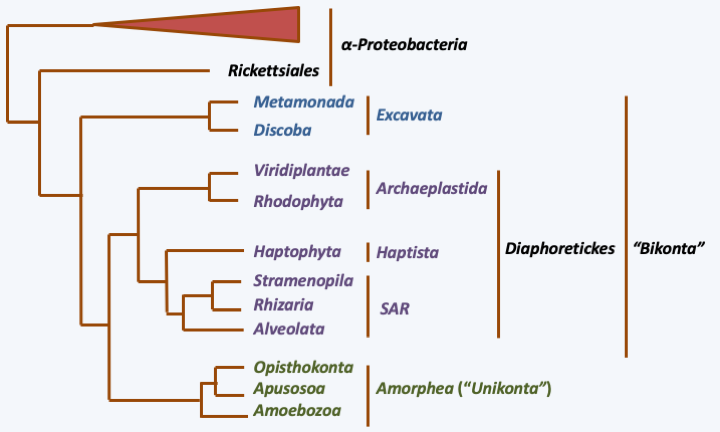
Figure 33.
Phylogeny of eukaryotes based on the sequences of 42 mitochondrial proteins.Back to chapter index