Archaeplastida
Back to main indexJump to section:
Introduction
The Archaeplastida super-phylum includes the direct descendants of the eukaryote that was the result of primary endosymbiosis of the plastid. Archaeplastida are therefore characterized by the presence of plastids surrounded by two membranes, exclusively providing them with energy for metabolism. They therefore generally no longer perform phagocytosis. Only a few prasinophytic algae, such as Cymbomonas, seem to have retained this characteristic. On the other hand, a substantial part of Archaeplastida retained the ability to move, thanks to the two anterochontic flagella typical of the Diaphoretickes. The almost constant presence of a cellulose-based wall surrounding the cell, on the other hand, prevents amoeboid movements. The monophyly of the group is confirmed by phylogenies based on plastid and mitochondrial DNA, but not always by nuclear genes. Analysis of the genes in the nucleus is probably complicated by the fact that many of them originate from transfer from plastids to the nucleus. Estimates indicate that, depending on the species of Archaeplastida, five to twenty percent of the nuclear genes come from the cyanobacterial symbiont and that these are not necessarily involved in the physiology of the plastid. In addition, other transfers have taken place from other bacteria, including Chlamydiae, further obscuring the evolution of the group. The lineage is known to be old, because the oldest fossils clearly belonging to eukaryotes are Archaeplastida, and more precisely Rhodophyta (see Figure 216). This places the event of primary endosymbiosis earlier than 1,200 million years ago. Since then, the Archaeplastida have diversified to occupy many ecological niches, obviously mainly those where light is available. Currently three branches of varying importance are recognized (Figure 217).
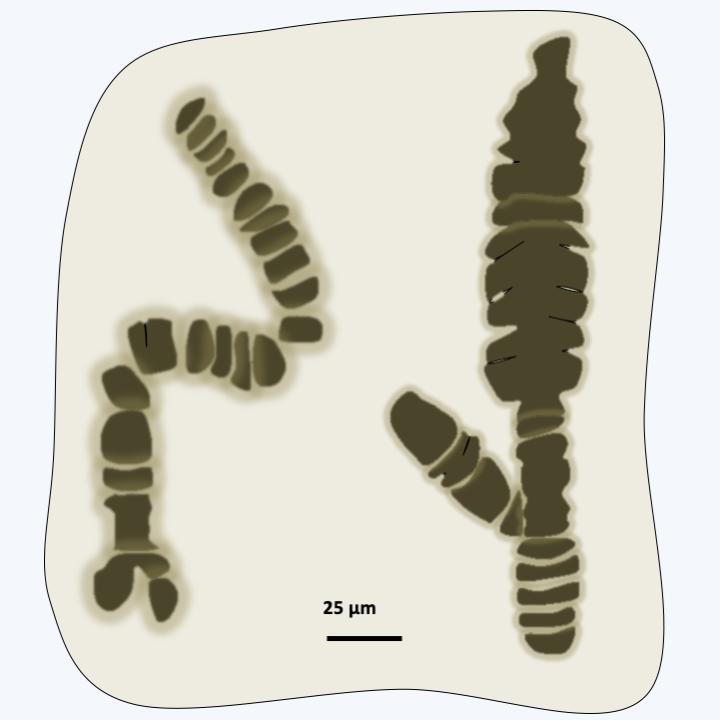
Figure 216.
Fossils of Bangiomorpha pubescens. These 1,200 million year old fossils resemble the present day Rhodophyta of the genus Bangia. These are the oldest fossils which are unquestionably eukaryotic.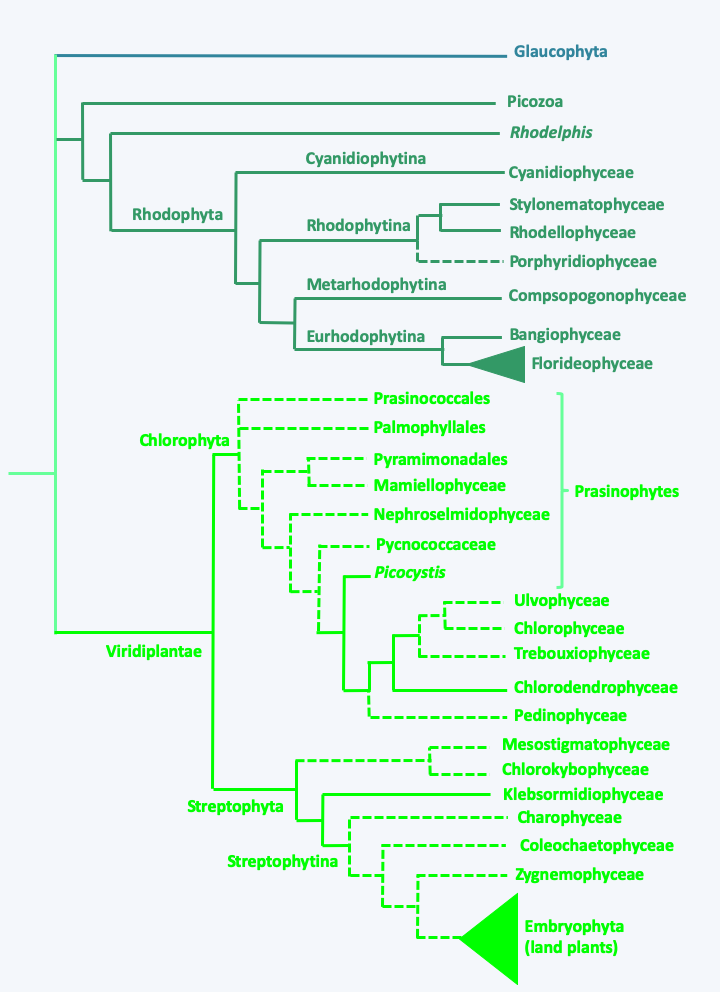
Figure 217.
Phylogeny of Archaeplastida. Note that Rhodelphis and Picozoa are non-phptosynthetic.Glaucophyta
The Glaucophyta phylum includes about twenty unicellular or colonial species (Figure 218) which appear to the sister group to the remaining Archaeplastida (Figure 217). Glaucophyta are rare in nature and live in fresh water, in free or fixed forms. They have submembrane alveoli, a character that they share with the Alveolata, without it being possible to determine whether this is a homology or a character resulting from convergent evolution. Some Glaucophyta have two flagella while others do not. The structure of the cell wall depends on the species, and can be bare, with a non-cellulosic wall or with a cellulosic wall. The most remarkable character of the Glaucophyta is their particular plastids, called muroplasts or cyanelles, which retain a trace of peptidoglycan between the two membranes (Figure 34). In the inner membrane, a simplified bacterial secretion system is present. All of its subunits, however, are encoded by nuclear genes. Plastid DNA is located in the center of the plastid in a structure that resembles a nucleoid. The cyanelle therefore strongly resembles the original endosymbiotic cyanobacterium in its structure. It is essential for the life of the algae because if the plastids are killed (by adding an antibiotic) the algae dies quickly. Besides chlorophyll a, the photosynthetic apparatus contains blue/green pigments of phycocyanin and allophycocyanin, giving a beautiful turquoise color. The carbon reserves produced are starch which is stored outside the plastid. The analysis of the enzymes involved in the synthesis of these reserves shows that their properties are similar to the enzymes found in the Rhodophyta. Both ribulose 1,5-biphosphate carboxylase (RuBisCO) subunits are encoded by the plastid genome.
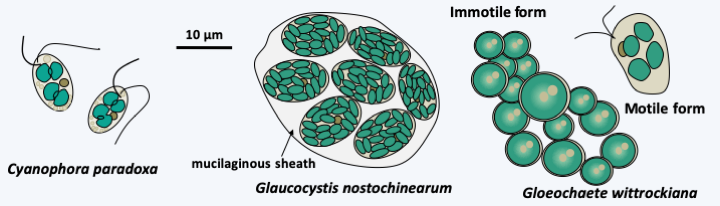
Figure 218.
Some Glaucophyta.Rhodophyta
The Rhodophyta or “red algae” branch brings together around 7,000 species. More than 95% of the species are marine and live in all the seas of the globe, where they constitute a significant part of coastal algae. However, they have also invaded terrestrial and freshwater biotopes. There is even one species, Rufusia pilicola, which grows specifically on the hair of three-toed sloths. Some have adopted a parasitic lifestyle with other Rhodophyta as hosts. Others live in symbiosis with marine protists such as Foraminifera. This may be the foreshadowing of additional secondary endosymbiosis, as Rhodophyta endosymbiosis have given rise to many other lineages of algae (see page 59 forward). Rhodophyta have very varied morphologies, because some are unicellular and measure 2 μm while others are multicellular and form thalli that can measure up to 50 centimeters. Between these two extremes, there is a whole set of species with thalli in the form of filaments, feathers, leaves, etc. They are most often sessile and hung via a “crampon” on the rocks or are more rarely planktonic. Some species, “coralline algae”, are calcified by a deposit of calcium carbonate in their wall. They form a very large proportion of the mass of coral reefs; in fact in some places they predominate over corals for reef construction! Similar calcified structures have been found in ancient strata dating from the Precambrian around 1,250 Ma ago. Finally, some species are able to endure particularly hostile biotopes such as low pH, heavy metals and/or hot water. For example, Cyanidium calderium has a growth optimum at pH = 2 and at a temperature of 57 °C!
The Rhodophyta have many shared characteristics, for example, they lack flagella. Other shared characteristics are that the small and large subunits of RuBisCO are encoded by the plastid, and that they lack glycosylphosphatidylinositol (GPI) anchors and autophagy. Their nuclear genomes seem to encode a reduced number of genes, because even a multicellular Rhodophyta like Chondrus crispus has only 9,600 genes. This figure is to be compared with the 27,000 genes of the land plant Arabidopsis thaliana and the 14,000 of the green algae Chlamydmonas reinhardtii. Each cell function appears to be encoded in the genome by single copy genes. The cell wall is made up of cellulose associated with other polymers, such as pectin, agar, carrageenans… Plastids have a linear genome. These contain many pigments including chlorophyll a and d, carotenes, and especially phycoerythrins and phycocyanins. The relative proportions of these last two pigments give the Rhodophyta their varieties of coloring. Indeed, the “red algae” are not all “red” but also sometimes brown, green or blue! The presence of these pigments allows the absorption and use of light waves that penetrate deep into the water (green, blue and purple light) thus supporting the growth of these algae to a depth of 100 m. The record is 268 m deep, where the light intensity is 0.5% of that of the surface. The main reserve polymer is Floridean starch or rhodamylon, a type of starch that is produced by polymerization of about fifteen glucose residues; this is stored outside the plastid.
In multicellular Rhodophyta, the separation of the two daughter cells after mitosis is often partial. Pores persist in the wall between the two newly formed cells and vesicles from the endoplasmic reticulum come to position in the opening (Figure 219). These intercellular junctions are then often sealed by protein plugs called “synapses”. The shape of these synapses serves as a phylogenetic marker. When cells of the same species are close to each other, they can engage in fusion processes not unlike the anastomoses of fungi (Figure 220). These fusions allow, among other things, to repair wounds and to ensure the cohesion of the thallus. In some species, the cells are plurinucleated, as in the syncytial mycelia of Eumycota. These common properties of thalli of Rhodophyta and Dikarya, as well as similar modalities of sexual reproduction (see legend forFigure 225andFigure 228), led 19th century biologists to postulate that Rhodophyta were the ancestors of fungi. We now know that these properties are evolutionary convergences. Reproduction can be asexual, via spore production or thallus fragmentation, or sexual depending on the different lineages. Currently, molecular phylogenies differentiate four sub-branches, one of which, that of Eurhodophytina, contains 98% of the species.
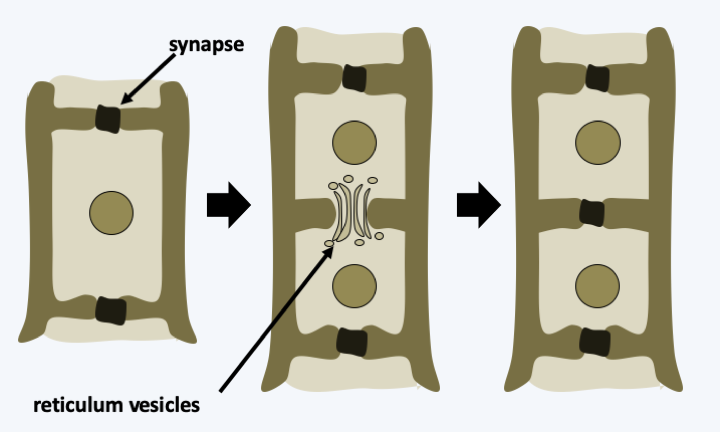
Figure 219.
Formation of a synapse.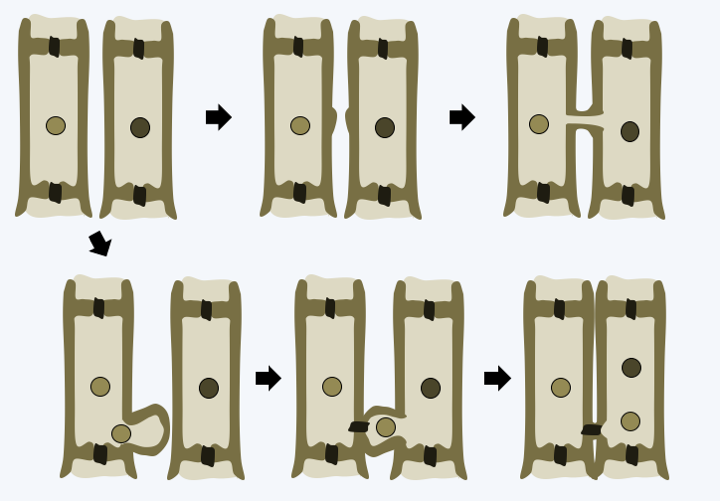
Figure 220.
Cellular fusion in Rhodophyta.Cyanidiophytina
The first branch of Rhodophyta that diverges, that of Cyanidiophytina, contains a few unicellular species that generally live in extreme environments such as hydrothermal vents (Figure 221). The genome of these algae seems even smaller than that of other Rhodophyta, because in Cyanidioschyzon merolae it contains only 5,300 genes. This species, like the other members of the group, is also very small, measuring only 2 μm at its greatest width. Besides its nucleus, this alga has only a mitochondrion, a plastid and a Golgi apparatus with two dictyosomes.
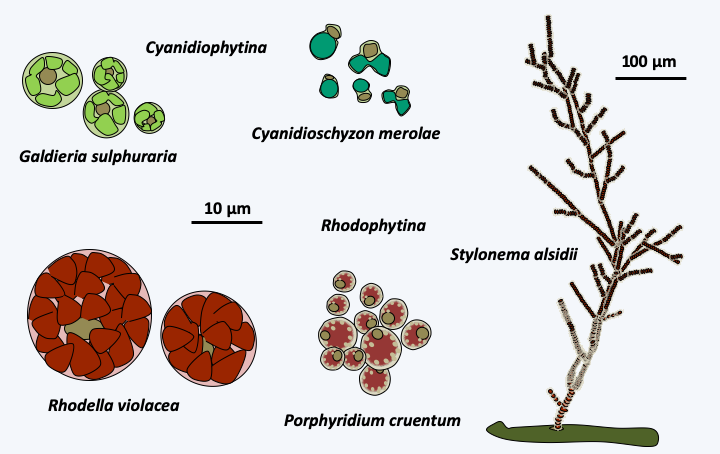
Figure 221.
Some Cyanidiophytina and Rhodophytina.Rhodophytina
The Rhodophytina subphylum contains a few dozen species that are unicellular or form microscopic filamentous colonies (Figure 221). The cells within these colonies are not connected by synapses. Unlike Cyanidiophytina, these algae are often marine and planktonic. Nevertheless, a few multicellular species such as Stylonema alsidii live fixed or have invaded the aerial environment, such as Rufusia pilicola inhabiting the fleece of sloths. The species of these two sub-phylum have no known sexual reproduction but some are capable of forming resistance spores.
Metarhodophytina
The third subphylum, that of Metarhodophytina, also brings together a few dozen species of colonial or multicellular algae, some of which are macroscopic (Figure 222). In a fraction of the species, those belonging to the orders of the Rhodochaetales such as Rhodochaete parvula and the Compsopogonales such as the Compsogon, the cells are connected by simple synapses (Figure 223). In contrast, in species of the order Erythropeltidales, such as Smithora naiadum, synapses are absent. Reproduction is still mainly asexual. However, a sexual process with production of male and female gametes has been detected in some species such as Smithora naiadum, although the entire cycle has yet to be established.
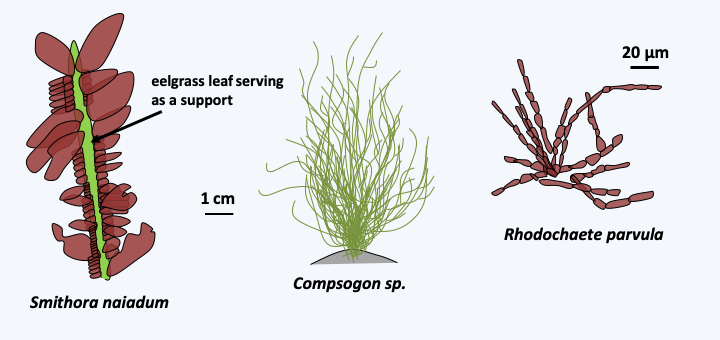
Figure 222.
Some Metarhodophytina.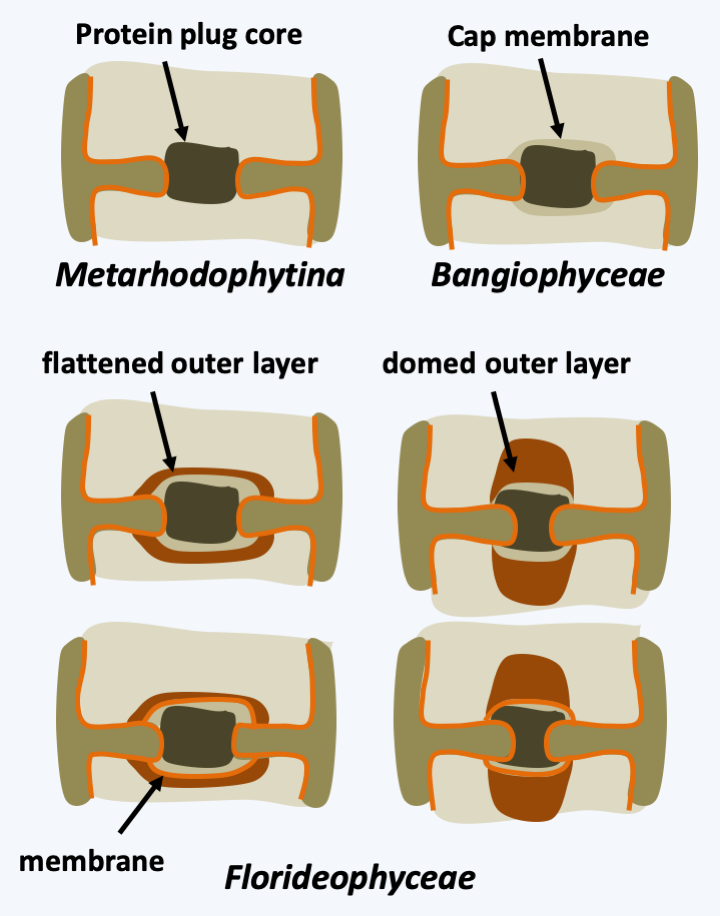
Figure 223.
Synapse structures in multicellular Rhodophyta. Only a few representative structures are shown for Floridaophyceae.Eurhodophytina
The last subphylum, that of Eurhodophytina, is divided into two classes (Figure 217), unequal in the number of species they contain.
Bangiophyceae
The Bangiophyceae class contains only about 200 species and only one order is the Bangiales. These algae are multicellular, but the multicellularity is still simple, without extensive differentiations. In Porphyra, the thallus is made up of a single layer of identical cells, and in Bangia, the filaments are made up of poorly differentiated cells (Figure 224). They have a haplo-diplobiontic type life cycle with two diploid stages and a haploid stage with very different morphologies, called the trigenetic cycle (Figure 225). Indeed, after fertilization, the zygote divides to give a carposporophyte, which remains on the haploid thallus and where carpospores, called zygotospores in Porphyra, will be produced. These will germinate to give rise to the second diploid stage known as “conchocelis”, because before phycologists discovered that it was only a stage of the life stage, it was classified in a separate genus, Conchocelis. The name comes from the fact that the conchocelis grows specifically on mollusc shells. They come in the form of microscopic branched filaments. The haploid thalli are of various shapes, filamentous or leafy depending on the species (Figure 224). Synapses are only present during the conchocelis stage and have a still simple morphology (Figure 223). It is in this order that we find the Bangia which resemble the fossils of Bangiomorpha (Figure 216andFigure 224) and especially the Porphyra which are used for human consumption. They are particularly appreciated in Asia for their nutritional value in vitamins and proteins and have been cultivated intensively for more than 400 years in Japan and 1,000 years in China. Porphyra is used to make “nori”. This product represents a market which amounts to hundreds of millions of euros annually; for example, in 2008 the market was around 1 billion euros for 110,000 tonnes of produced seaweed. Note that the elucidation of the Porphyra life cycle in 1949 was important (Figure 225) because it allowed the control of cultures.
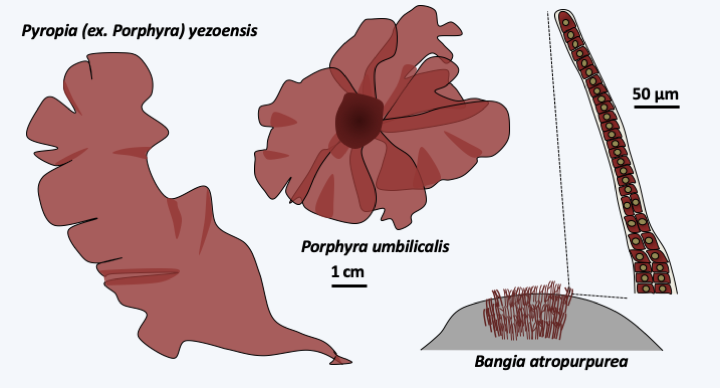
Figure 224.
Some Bangiophyceae.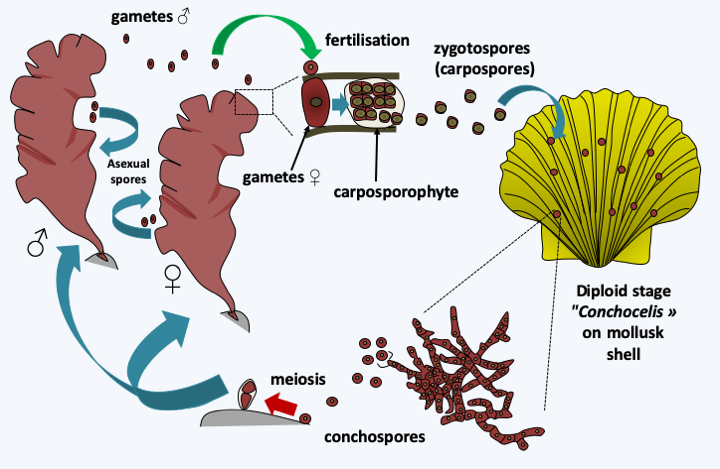
Figure 225.
Porphyra cycle. The cycle begins with the germination of the conchospores which is accompanied by meiosis to give rise to male or female haploid thalli. In aquaculture, it is these spores that are used to initiate the cultivation of algae. Some species, but not all, make asexual spores which are sometimes used for this purpose. Haploid thalli differentiate between male and female gametes according to their sex. Male gametes fertilize female gametes to give rise to a zygote which divides several times. The resulting carposporophyte will produce zygotospores, also called carpospores. The zygotospores will germinate on the shells of marine molluscs and give rise to the conchocelis thalli which will produce new conchospores.Floridaophyceae
The Floridaophyceae class of Eurhodophytina is the most diverse, currently grouping together nearly 7,000 species. Floridaophyceae are very common in coastal areas, where they play important roles as primary producers. These Rhodophyta are multicellular and show a wide variety of shapes, ranging from filaments to more or less branched plumes or laminae (Figure 226). The cells are linked by complex synapses whose morphology varies according to the different orders of the class (Figure 223). Thalli are often complex. For example, in columnar thalli, the central cells are large in size and branch out into smaller cells, which repeat the process. The cells then remain attached to each other to form a central medulla and a peripheral cortex (Figure 227). Growth is usually apical but may be intercalary in a few families. Some cells differentiate to secrete different brominated, iodinated or mucilaginous products. For the most part, Floridaophyceae have a complex trigenetic life cycle (Figure 228). In some orders the two diploid stages are very different, as in Bangiophyceae and in others they have similar morphologies. Likewise, significant variations exist over the duration of these three stages, some of which may be very reduced, or even non-existent in certain species. It is to this group that the calcified coralline algae belong, and several species are used to produce important compounds: agar (or agar-agar) and carrageenans. These compounds used mainly by the food industry are polymers of sulfated galactoses. With two other polymers, funorane and porphysane, their presence serves as protection for the algae to prevent too rapid desiccation. The use in laboratories of agar in Petri dishes is well known. It is also used in food preparations such as jellies or desserts. Carrageenans are used as thickening agents in various culinary preparations including milkshakes, ice creams, yogurts, custards and various creams. Funorane, which is similar in composition to agar, is used as a glue in some industries in Japan and China. The current markets for these products, combined with that of alginates produced by brown algae, are significant, amounting to over half a billion euros. Coralline algae are also used as a soil conditioner and to amend calcareous soils. Maerl is a mixture containing these algae, which is dredged from the ocean floor, crushed to a powder and used as an agricultural fertilizer. It is rich in mineral salts. Its addition to the soil, up to 500,000 tonnes per year in France, makes it possible to enrich the soils and prevent their acidification. The slow growth of maerl makes its use controversial, as there is no way for the algae to regrow to the same pace as it is being harvested.
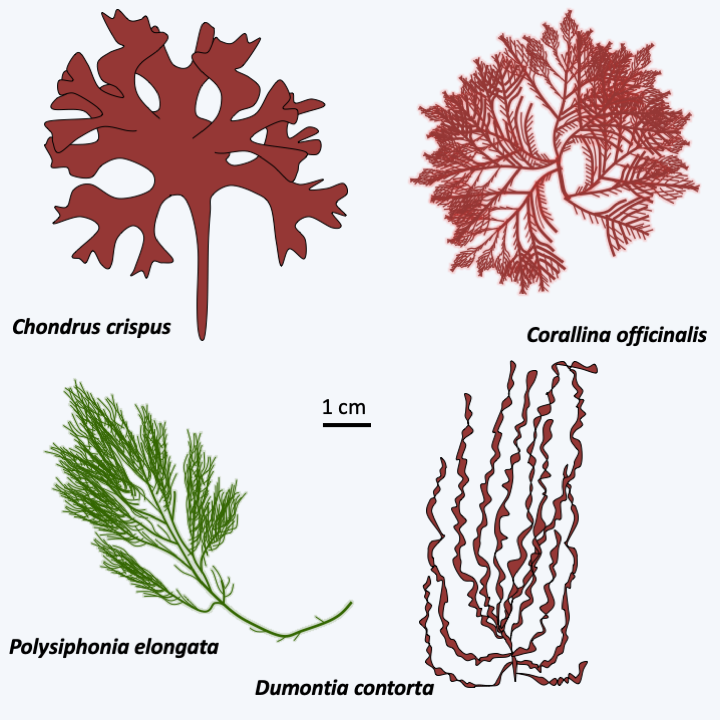
Figure 226.
Some Floridaophyceae.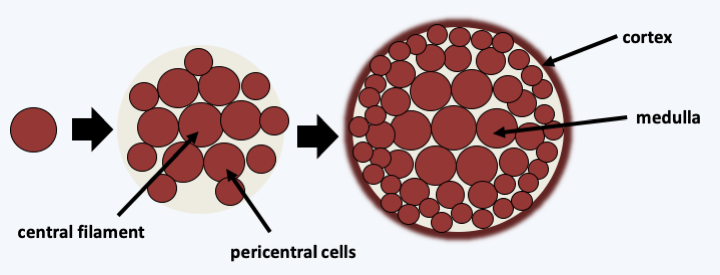
Figure 227.
Typical structure of a cylindrical thallus of Floridaophyceae.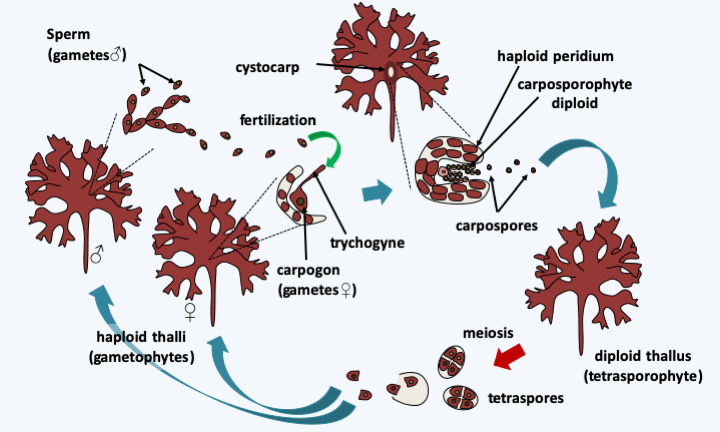
Figure 228.
Cycle of Condrus crispus. The cycle begins with the germination of tetraspores resulting from meiosis to give rise to male or female haploid or gametophyte thalli. These differentiate according to their sex either male gametes or sperm, or female gametes or carpogonia. Male gametes released into the water will fertilize female gametes by fusing with trichogyne, a threadlike extension of the female gamete. The zygote resulting from fertilization divides several times, protected in a structure carried by the maternal thallus, called a cystocarp. The carposporophyte thus obtained will produce the carpospores. The carpospores will germinate to give rise to diploid or tetrasporophyte thalli in all points similar to haploid thalli. This cycle superficially resembles that of Pezizomycotina. Indeed, the tetraspores resemble tetrads, the haploid thalli which result from them produce gametes of morphologies similar to those of certain classes of Pezizomycotina such as the Sordariomycetes, and fertilization is also followed by a stage of cell divisions in a structure whose peridium has a maternal origin. The analogy ends here in the case of Condrus crispus, because plasmogamy is immediately followed by karyogamy and two diploid thalli are differentiated before meiosis, while in Pezizomycotina a long dikaryotic stage is present; moreover, this is immediately followed by meiosis. In some other Floridaophyceae, the tetrasporophyte is reduced, increasing the similarity of cycles.Viridiplantae
The third and last major branch belonging to the Archaeplastida, that of the Viridiplantae, is the most diverse. It contains algae, called “green algae”, but also land plants. The land plants (or just plants; Embryophyta) emerge as a monophyletic group from within one of the two currently recognized sub-branches of Archaeplastida, that of Streptophyta. Green algae are however paraphyletic, being spread out over many different lineages of Archaeplastida (Figure 217). There are approximately 11,000 species of green algae and over 250,000 species of plants. However, green algae have a much greater genetic and biological diversity than plants. Most species occupy freshwater, but part of the Chlorophyta algae lives in the sea, either attached to a substrate or as coastal plankton. A few green algae have adapted to life on earth in humid areas such as tree trunks, wet soil or melting snow. Some proliferate in the deserts. Many live in symbiosis such as chlorellae or lichen phycobionts. They can be unicellular and mobile, most often thanks to two anterochontic flagella, or else immobile and without flagella. These unicellular algae can be very small, like some Prasinophyta which do not reach 2 μm in diameter, while species of the genus Acetabularia can reach 10 cm in height, while remaining uninucleated. Multicellularity, which is exclusively non-aggregative, has emerged multiple times during the evolution of Viridiplantae. While it has reached its greatest complexity in the Embryophyta, there are however many other colonial or multicellular lineages, whose forms range from simple filamentous thalli to thalli in the form of leaves, plumes, spheres… In each of these lineages, the different forms appeared by convergent evolution, which prevents the use of morphological criteria in phylogeny, at least to define the major lineages (see for example Figure 57: the evolution of Chlamynomonadales / Volvocales). In some cases, multicellularity results from the assembly of cells such as in plants, but in other cases, algae are unicellular and multinucleated syncytia. Apart from the presence of plastids from primary endosymbiosis, these algae have few shared characters. The plastid, surrounded by two membranes, contains chlorophyll a and b, as well as accessory pigments of the carotenoid and xanthophyll types. These pigments are suitable for collecting low penetrating red and blue lights, explaining the distribution of these algae exclusively on the surface of bodies of water, with the exception of Palmophyllales which can be found up to 100 m deep. Synthesis of the reserve polymer, starch, takes place in the plastid and the small subunit of RuBisCO is encoded by the nuclear genome, unlike Glaucophyta and Rhodophyta. Most Viridiplantae algae have a cellulose-based cell wall, which is pierced with plasmodesmas in many lineages, but some species are bare or covered with organic scales. The diversity of their forms and biology of Viridiplantae algae indicate an ancient history, even though the earliest known fossils are only 750 million years old. This ancient history is confirmed by a great divergence of their genomic sequences, making a conclusive phylogenetic tree problematic to establish (Figure 217). However, the classification into two major sub-branches, Chlorophyta and Streptophyta, initially based on the structure of the flagellum base, the presence of a cell wall and the modalities of mitosis, are largely confirmed by molecular phylogenies (Figure 217).
Chlorophyta
The Chlorophyta clade contains about 6,000 species. The evolution of the group begins with the rapid separation of several lines of single-celled flagellated algae, often very small, collectively grouped into the class Prasinophyceae (Figure 229). However, the flagellum has been repeatedly lost and several unrelated Prasinophyceae are coccoids. Likewise, the Palmophyalles form colonies composed of coccoid cells embedded in a gelatinous matrix which covers the rocks in places poor in light, thus showing the beginning of multicellularity. The cells are bare or covered with organic scales. Sexual reproduction seems to exist but is difficult to demonstrate. Some genera, like Cymbomonas or Micromonas, are able to phagocytize bacteria, supporting a phagotrophic origin for Archaeplastida. These bacteria serve as food supplements in the absence of light or trace elements, but cannot ensure the long-term survival of these algae, as the mixotrophic mode of nutrition seems obligatory. Most Prasinophyceae are marine but a few are freshwater. This class is formally destined to disappear because it is paraphyletic (“Prasinophytes” inFigure 217). Although containing only a few dozen morphospecies, at least seven lineages are currently defined in the class. Their order of branching remains obscure due to their great genetic and biological diversity. In addition, several lines are only known from environmental DNA. The cell morphology, the shape of the scales, the modalities of their division and the structure of the flagella are very varied, confirming an ancient origin of the different lineages. The best known lineage is that of Mamiellophyceae. This clade contains the Ostreoccocuses, planktonic algae that are marine or living in coastal lagoons. They are unicellular, coccoid and are among the smallest known eukaryotes because they only measure one micron in diameter. Their volume is therefore only one tenth that of Escherichia coli. Full-length sequencing analysis reveals a small, compact genome of around 12.5 Mb and a coding capacity of around 8,000 genes. Comparison of the genomes of Ostreococcus tauri and Ostreococcus lucimarinus indicates that these two species share only 95% of their genes and that the orthologous proteins only resemble each other with 70% identity. Such differences are similar to those observed between the genomes of fish and those of mammals… The genomes of different isolates of Micromonas pusilla, flagellated algae also belonging to Mamiellophyceae, show the same tendencies, suggesting that this morphospecies masks a wide genetic diversity. In fact, the genomes of different isolates growing in different biotopes seem adapted to their specific conditions. They therefore probably represent several different species with identical morphology. In contrast to these compact genomes, that of Cymbomonas tetramitiformis, belonging to the Pyramimonadales order, measures around 1,000 Mb and encodes more than 37,000 genes. Among these are genes known to be involved in phagocytosis, as expected from the mixotrophic mode of nutrition of these algae. The genome seems to be missing some genes involved in amino acid biosynthesis, suggesting that their absence prevents the definitive loss of phagotrophy in Cymbomonas. Among the genes present in the sequenced genomes of Prasinophyceae are those for peptidoglycan biosynthesis, the origin of which is probably cyanobacterial. The synthesis of peptidoglycan in these algae, as in the moss Physcomitrella patens which also have these genes, remains to be demonstrated.
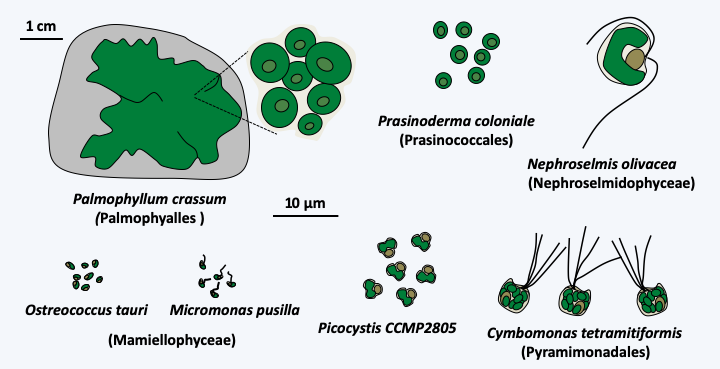
Figure 229.
Some Prasinophyceae.All of the other Chlorophyta or “core Chlorophyta” are monophyletic and are related to one of the lineages of “Prasinophyceae”. Currently, phylogenies suggest that Picocystis and related algae are sister to the “core Chlorophyta”. Two lineages, Pedinophyceae and Chlorodendrophyceae, currently having class status and formerly ranked with the other Prasinophyceae, diverged the earliest from the rest of the core Chlorophyta (Figure 230). Both classes comprise unicellular marine or freshwater algae. Pedinophyceae have a single flagellum and lack cell walls or other protective structures. There are about twenty species in this class. Their exact phylogenetic position within the “core Chlorophyta” is still unknown, but their mode of division, which does not involve a phycoplast, suggests that they may have diverged first as shown inFigure 217. The phycoplast is a network of microtubules which forms parallel to the plane of division and participates in defining the site of cleavage (Figure 231). Chlorodendrophyceae have four flagella and are protected by fused organic scales. There are about fifty species. They also use a phycoplast during division. Molecular phylogenies clearly place them as a sister group to Trebouxiophyceae, Chlorophyceae and Ulvophyceae, the other three classes of “core Chlorophyta”. However, the branching order of these three classes is still debated and varies depending on the genes used to define phylogenies.
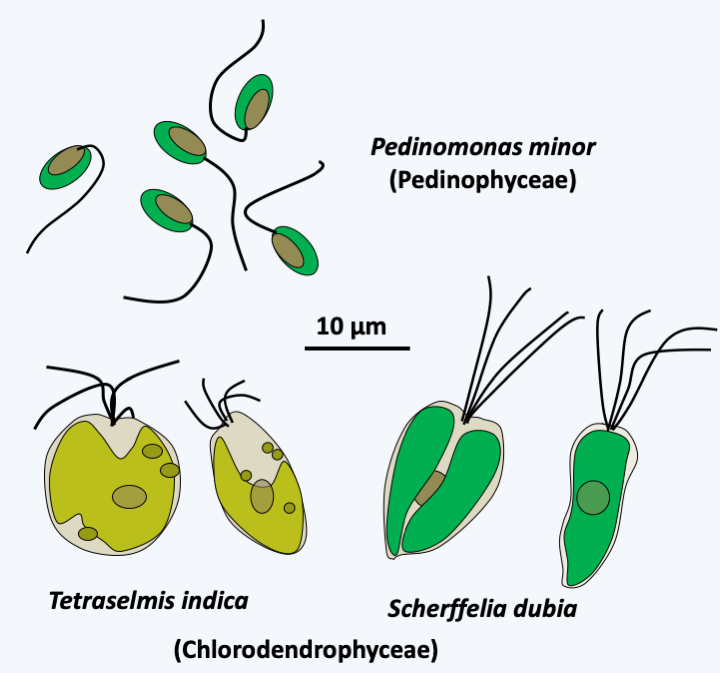
Figure 230.
Some Pedinophyceae and Chlorodendrophyceae.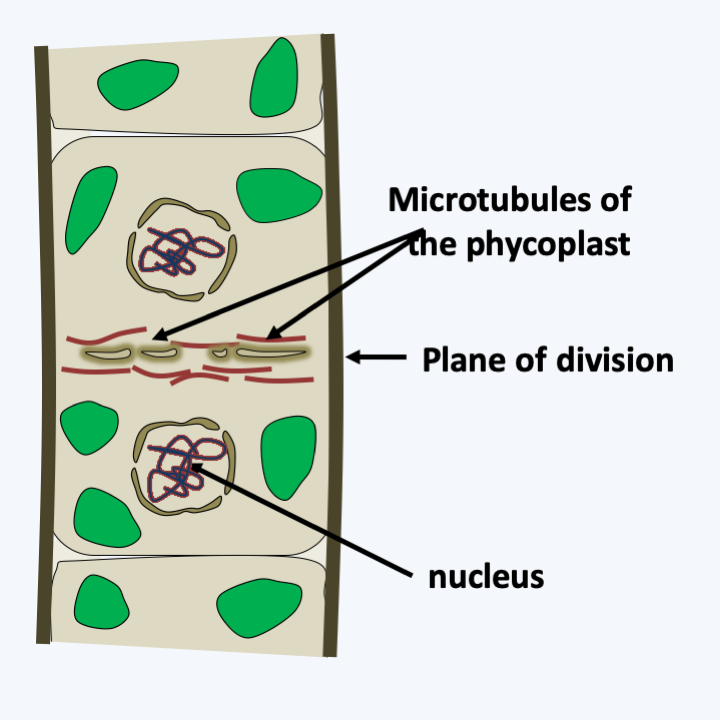
Figure 231.
Division with phycoplast.Chlorophyceae
The class of Chlorophyceae is the numerically largest with more than 3000 described species (Figure 232). They are mainly freshwater with some terrestrial species. Chlorophyceae are unicellular or form simple multicellular thalli: filaments and spheres of varying complexity. Some are motile and use two or four flagella, others are immobile. Several species have, like the Euglenida, a light sensitive organ called stigma. This primitive “eye” is the simplest and most common system for detecting light. It contains rhodopsin as a photoreceptor, as in animals. After activation of the photoreceptor by light, a Ca+2 channel is activated, which causes a massive influx of calcium into the region exposed to light. At low light levels, the generated depolarization specifically activates the beating of the flagella, which changes the direction of swimming. In continuous light, this causes a positive phototropism towards the light source. If a flash of bright light is given, it causes a massive influx of calcium into the eye, conversely causing a phobic response to light. While most Chlorophyceae are exclusively photoautotrophic, species of the genus Polytomella live in waters rich in organic matter and have lost photosynthetic capacity. The type species of the Chlorophyceae are Chlamydomonas reinhardtii (Figure 233) and Volvox carteri (Figure 234). Chlamydomonas and Volvox are model systems for studying molecular genetics in several laboratories. The first is by far the most studied because techniques have been developed for a longer time than in the latter. Chlamydomonas, being unicellular, haploid with a well-defined cycle, is easily manipulated. For example, it is possible to easily isolate many mutants and perform classical genetic analyzes, but also to inactivate genes and perform reverse genetics analyzes. It is used to study, among other things, photosynthesis or the establishment of flagella and stigma. In Volvox, the asexual phase is more complex. Indeed, this organism has a simple multicellular structure with differentiations between a “somatic” line comprising a single type of cell and germinal line also comprising a single cell type, as well as simple morphogenetic processes. It serves as a model to study the evolution of multicellularity. The complete sequences of the genomes of these two algae are available. The genomes are similar in size and composition, despite the different structures of the two algae, confirming that the transition to multicellularity requires little genetic invention. Indeed, the Chlamydomonas genome contains 14,516 genes distributed over 118 Mb of sequence and Volvox 14,520 genes over 128 Mb.
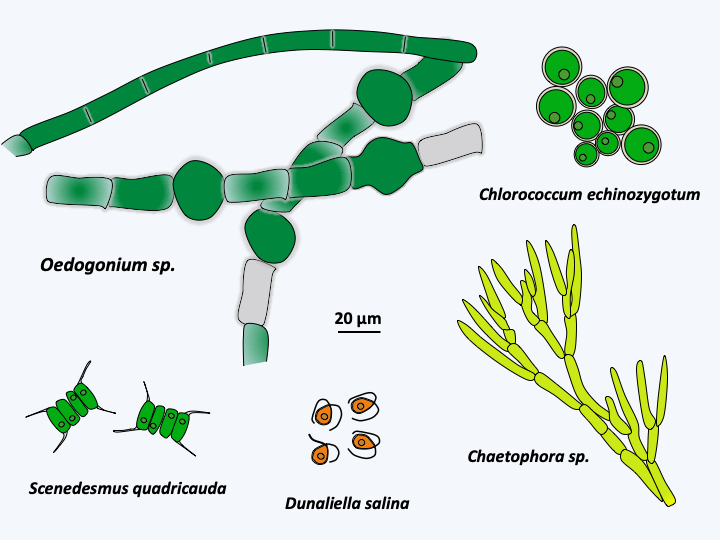
Figure 232.
Diversity of Chlorophyceae.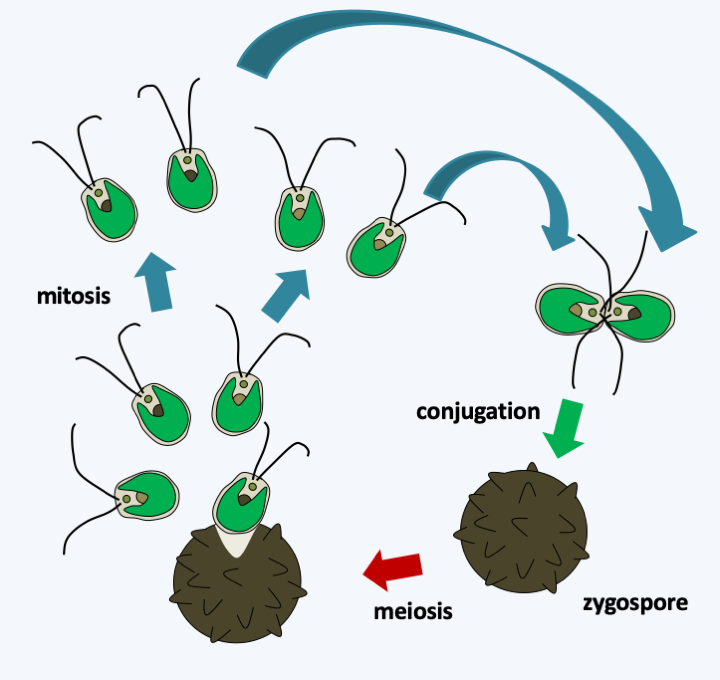
Figure 233.
Cycle of Chlamydomonas reinhardtii. Like all Chlorophyta, this alga has a haplobiontic cycle. The reduced diploid phase often presents as a form of resistance or zygospore. At the end of the meiosis, four spores are formed from the zygospore, which allows an analysis of tetrads as in Ascomycota yeasts. The algae is bipolar heterothallic. Plastid is transmitted from one parent and mitochondria from the other.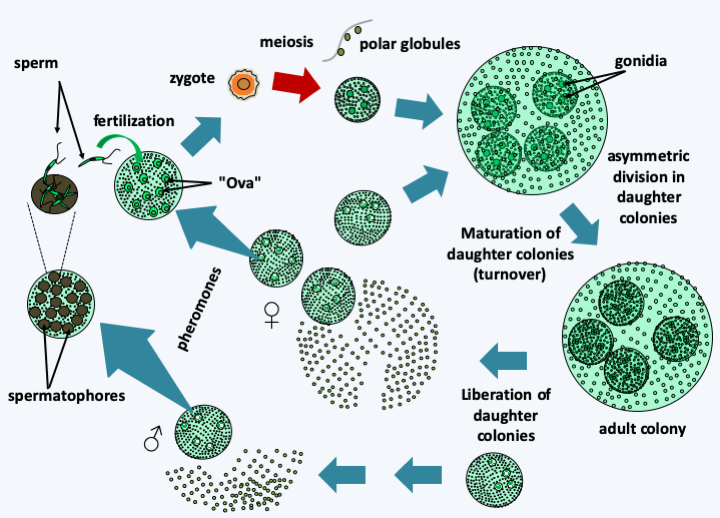
Figure 234.
Volvox carteri cycle. In adulthood, colonies consist of 2,000 chlamydomonas-like biflagellate cells and 16 germ cells or gonidia. The synchronized movements of the somatic cell flagella allow movement of the entire colony. Differentiation into somatic and germ cells is related to asymmetric cell division, with the large cell becoming the germ cell. During maturation, the gonidia move inside the colony, make four mitoses and then asymmetric divisions; mini-colonies therefore develop in the main colony. Daughter colonies have flagella facing inward. To correctly orient the flagella, an inversion occurs like that of a thermowell. Then, the mother colony tears itself apart and frees the daughter colonies. Volvox carteri can also reproduce sexually, during which gonidia subjected to sex pheromones differentiate into colonies carrying large 'ova' or biflagellate 'sperm' of smaller size and clustered in spermatophores. There are therefore male and female colonies under the control of a complex bipolar sex locus. Fertilization leads to a zygospore which at the time of germination causes meiosis. This produces a single viable cell, either male or female, and three polar cells which abort. The viable cell divides and differentiates into a colony.Trebouxiophyceae
The Trebouxiophyceae class is rich with more than 800 species. They are unicellular algae, filamentous or with more or less organized thalli (Figure 235). A number of these algae are microscopic in size and are among the smallest known eukaryotes, such as Nanochlorum eukaryotum which exhibits intriguing characteristics. It measures 1.5 μm and has a single plastid and a single mitochondria. Interestingly, it appears to be devoid of histones and nucleosomes, which calls into question how it partitions its nuclear genome at the time of mitosis. Most species reproduce primarily asexually. In species where sexual reproduction is known, the life cycles are of the diplobiontic or haplodiplobiontic type. Trebouxiophyceae have colonized most environments including humid aerial regions such as tree trunks, etc. Many species live in mutualistic or parasitic symbiosis. The “chlorellae”, most of which are Trebouxiophyceae and a few Chlorophyceae, live in endosymbioses with animals. Species of the genera Trebouxia, Pseudotrebouxia, Myrmecia, etc. are frequently associated with fungi to form lichens. The few parasitic Trebouxiophyceae have lost the ability to photosynthesize. Helicosporidum parasiticum attacks insects. It penetrates insects by ingestion in the form of unicellular cysts and is found in filamentous form in the hemolymph of the insect. In this medium, it proliferates and generates spores which themselves generate cysts, which are excreted in the medium after perforation of the insect’s cuticle. Similar species to Helicosporidum parasiticum belonging to the genus Prototheca are opportunistic pathogens in humans in whom they cause protothecosis and in cattle and dogs in which they cause mastitis. These algae are colorless, round and without flagellum. They are ubiquitous in nature and live in soils where they consume various organic materials. Prototheca wickerhamii is the pathogenic species of humans; Prototheca zopfii is the pathogenic species of animals. They have the ability to colonize the digestive tract without causing disease. In animals, Prototheca zopfii can invade various organs and thus cause death. In humans, Prototheca wickerhamii causes dermatitis, localized infections following infected wounds or systemic infections in immunocompromised patients. An extracellular polymer based on sulphated galactan is probably responsible for the inflammations caused by these algae. Molecular analyzes show a complex evolution of Trebouxiophyceae with evolutionary convergences both in terms of morphology, loss of flagella and styles of life.
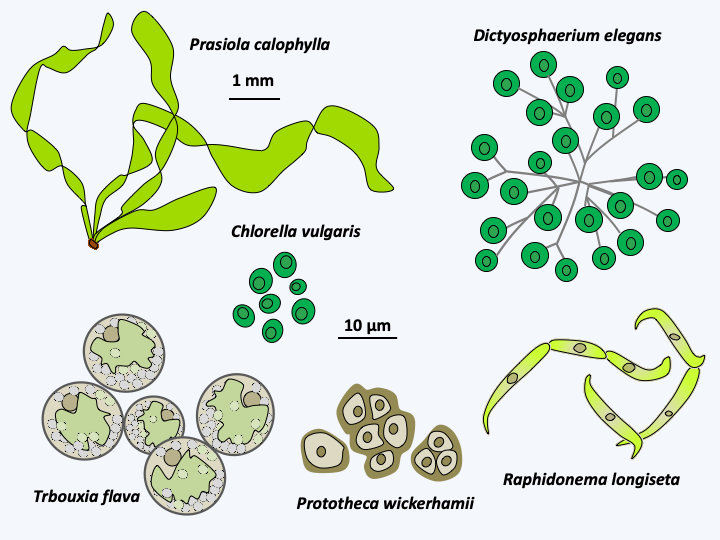
Figure 235.
Some Trebouxiophyceae.Ulvophyceae
The last class of the “core Chlorophyta”, that of Ulvophyceae, contains nearly 2,000 species. They are mainly marine and sessile, often forming large thalli. Some are found in fresh water or in humid aerial environments. Some, like the Trentepohlia, live as lichen phycobionts. Ulvophyceae are well known to the general public because several species are consumable, commonly known under the name sea lettuce. The thalli can be filamentous, in the shape of lettuce leaves or intestines, or more complex (Figure 236). They can be unicellular, with sizes ranging from microscopic to more than 10 centimeters in height, or multicellular. In the latter case, the thallus can be composed of uninucleated or plurinucleated cells and therefore present a coenocytic structure. Some species are even completely devoid of partitions and the thallus is a giant “siphon”, possibly measuring several centimeters. This is the case, for example, with Bryopsis plumosa, an alga that participates in reef ecosystems. The thallus is aseptate with a vacuole that occupies the center of the cell and a thin layer of cytoplasm at the periphery, this structure allows the rapid exchange of organelles, nutrients and other metabolites that are found within the same cytoplasm. However, this type of organization makes the algae very vulnerable to physical attacks. Indeed, the lack of a septum makes it impossible to isolate a damaged compartment. Bryopsis plumosa has therefore developed a system that allows the regeneration of a cytoplasmic wall under extreme conditions. When the algae is cut, it extrudes “sub-protoplasts” which are gelled assemblages of cytoplasm containing organelles and potentially nuclei. In about ten minutes, the sub-protoplasts generate a gelatinous mucus which ensures their protection. Then a groove forms between this gelatinous layer and the cell contents. At this stage, the sub-protoplasts can still fuse. After a dozen hours, a plasma membrane is regenerated and four hours later it is the turn of the cell wall to form. The gelatinous layer has the semi-permeability properties typical of plasma membranes. It is therefore a temporary alternative to the plasma membrane having the same properties. This type of siphoned algae is very efficient. In fact, the species Caulerpa taxifolia is currently invading the depths of the Mediterranean. This species is normally tropical. An “aquariological” variety that can live at a lower temperature was accidentally released from aquariums in Monaco. Since then, it has spread rapidly, destroying native ecosystems. Indeed, it thrives in autumn when the waters are very warm, while the other algae wither. With the return of spring, they then invaded the premises. Some claim that the algae synthesizes toxins which could then accumulate in the food chain and propose its eradication. The current focus is on the use of copper and herbivorous slugs, but success is variable. It seems, however, that after proliferating, it disappears on its own.
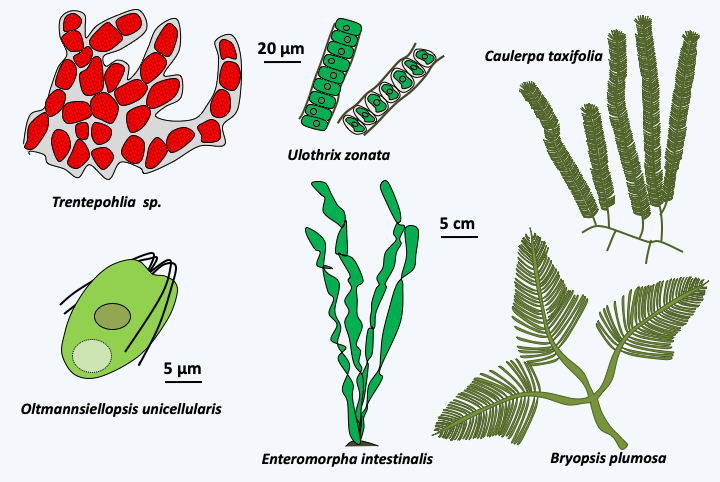
Figure 236.
Diversity of Ulvophyceae.Type species for Ulvophyceae are Ulva lactuca (Figure 237), a multicellular alga with a thallus composed of uninucleated cells, and Acetabularia acetabulum (Figure 238), a large unicellular algae. Species belonging to the genus Acetabularia have long served as laboratory models. It is in particular thanks to these algae that Joachim Hämmerling (1901-1980) showed that the genetic information which directs development is contained in the nucleus. The cells are made up of 3 parts: the rhizoidal base, the stem and the head which differentiates at the time of reproduction. However, the single nucleus is located in the base. Due to their large size, stem-to-base grafting experiments using species with different head morphologies are possible. In all cases where a head regenerated, it was of the type of the base containing the nucleus and not of the type of the stem…
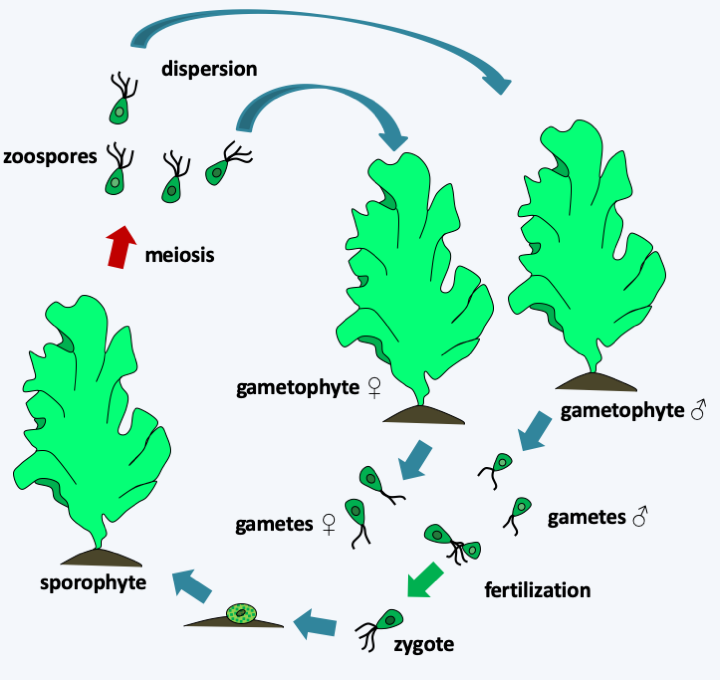
Figure 237.
Ulva lactuca cycle. In this species, the cycle is haplodiplobiontic. Diploid thalli, or sporophytes, and haploid, or gametophytes, have identical morphologies. Note that they are only two cells thick but can reach a size of over 30cm in height. There are male gametophytes and female gametophytes. Male gametes are slightly smaller than female gametes, but have the same morphology. All are biflagellates. After fertilization, the quadriflagellate zygote encysts and then differentiates from the sporophyte. This produces quadriflagellate diploid zoospores which restore male and female gametophytes.Once again, the evolution of these algae is complex with many evolutionary convergences at the morphological level and the appearance of multicellularity. However, coenocytic algae appear to be monophyletic, and siphoned algae are related to large unicellular algae such as Acetabularia and together form a monophyletic group, without it being possible to conclude whether these two types of organization have unique origins. Ulvophyceae have interesting interactions with bacteria which seem to participate in the establishment of multicellularity. The Enteromorpha and two related algae, Monostroma oxyspermum and Ulva lactuca, have been shown to use the thallusin produced by bacteria as a morphogenesis factor. In the presence of this molecule, they grow as characteristic thalli, while without the factor they grow as aggregates of undifferentiated cells! Species of the genus Enteromorpha are very common in the intertidal zone. Their motile zoospores attach to the substrate to differentiate a new individual, and are able to detach if the substrate is not suitable for them. The substrates they colonize are bacterial biofilms. In fact, they are able to detect N-acylhomoserine lactones, molecules used for bacterial quorum-sensing and participating in the establishment of biofilms.
Streptophyta
The second large clade of Viridiplantae, that of Streptophyta, encompasses both algae, together known as “charophytes” (a paraphyletic group), and land plants (Embryophyta), emerging as a monophyletic group from charophyte ancestors. The separation with the Chlorophyta is old, probably going back over a billion years.
Mesostigmatophyceae and Chlorokybophyceae
Two lineages appear to have diverged first, Mesostigmatophyceae and Chlorokybophyceae (Figure 217). The most recent analyzes suggest that these two classes form a monophyletic line, but this result is still debated. Both classes consist of unicellular algae with no known sexual reproduction. The only known species of Mesostigmatophyceae, Mesostigma viride (Figure 239), is interesting because it is the only species of Streptophyta having flagella during its vegetative phase, in the other members of the group, the flagellated forms serve only for dispersal or reproduction. These flagella are inserted laterally, which appears to be a shared trait of Streptophyta. In addition, this freshwater alga does not have a cellulose wall but is covered with scales and mucilage like Prasinophyceae, with which it has long been classified. Partial transcriptome analyzes show that Mesostigma viride presents photosynthesis characteristics similar to plants, such as the use of a particular plastidic glyceraldehyde-3-phosphate dehydrogenase, confirming its key phylogenetic position to understand the origin of land plants. The only species of Chlorokybophyceae known from other information than solely by DNA sequences, Chlorokybus atmophyticus, is a rare alga that lives on wet rocks, where it forms small cubic clumps of a few cells; it disperses using biflagellate zoospores (Figure 239). The cells are surrounded by a wall, the exact composition of which is still unknown, but which is different from those of plants because it is free of xylans, mannans or xyloglucans.
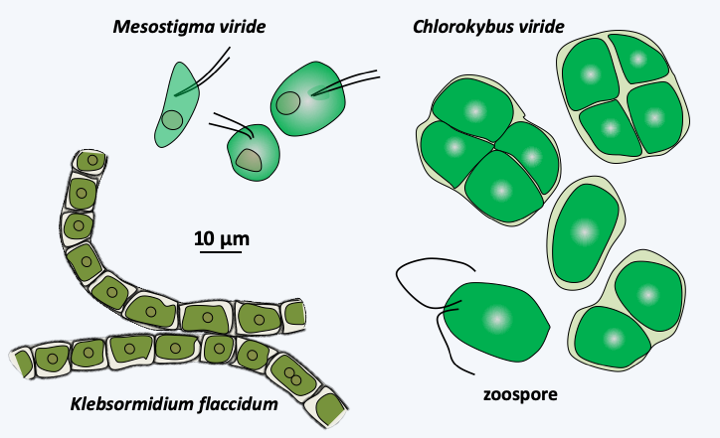
Figure 239.
Some early-diverging Streptophyta.Klebsormidiophyceae
A third line which has diverged after Mesostigmatophyceae and Chlorokybophyceae, that of Klebsormidiophyceae, also presents primitive characters. This class currently has about fifty freshwater and terrestrial species. They form single filamentous or clustered colonies (Figure 239) and reproduce, like Chlorokybophyceae, through the production of biflagellate zoospores. They can survive harsh conditions by producing akinetes, which are spores produced by thickening of the cell wall. However, this wall does not have the same structure as that of plants. Analysis of the Klebsormidium flaccidum genome has shown that this alga already has some of the characteristics that plants need to live in terrestrial habitats. It has the enzymatic equipment to produce several hormones used by plants for their development or to respond to stress such as auxin (indole 3-acetic acid), abscisic acid, isopentenyladenine, jasmonate or salicylic acid. Klebsormidium flaccidum has the receptors for these hormones, suggesting that it can use them to signal stress responses. Its plastid genome encodes the genes for one of the two systems used by plants to protect the photosynthetic apparatus during too much sunlight. This system, based on a complex of 18 proteins and resembling an NADH dehydrogenase, creates a cyclic flow of electrons. Intriguingly, it thus seems that the Klebsormidiophyceae have acquired some of the properties that have been necessary for plants to get out of water.
Charophyceae, Coleochaetophyceae and Zygnemophyceae
The other three classes of charophytes, Charophyceae, Coleochaetophyceae and Zygnemophyceae, present characteristics similar to the Embryophyta both in terms of the structure of their wall and the mode of cytokinesis. Indeed, some species synthesize mannans, xylans and xyloglucans and sporopollenin, or even lignin, like plants. However, the mechanisms of biosynthesis are not yet known, thus not allowing us to conclude whether these are homologies or evolutionary convergences. Cytokinesis follows the same procedure and uses a phragmoplast (Figure 240), whereas the Mesostigmatophyceae, Chlorokybophyceae and Klebsormidiophyceae divide by means of a groove like the protozoa. Most are freshwater algae, with a few species growing in brackish water. Phylogenies indicate that the Zygnematophyceae class would be closest to the plants and that of the Charophyceae the most distant, the Coleochaetophyceae class occupying an intermediate position (Figure 217). This branch order, which has not yet been definitively validated, is surprising because Zygnematophyceae algae have a much simpler thalli than those of Charophyceae and Coleochaetophyceae. This order would therefore suggest either numerous evolutionary convergences of Charophyceae and Coleochaetophyceae with plants, or, more parsimoniously, an evolution towards reduced complexity in Zygnematophyceae. With more than 3,000 validated species, this class contains a good part of the Viridiplantae algae which live on the bottom of fresh water, where they form an important part of the primary producers. These algae are devoid of flagella and therefore have reduced mobility. Most are unicellular, but there are filamentous or colonial species (Figure 241). In multicellular species, there are no plasmodesmas between cells. They often have surprising geometric shapes (Figure 241). Their multiplication occurs mainly through asexual divisions, which in certain species, such as Micrasterias, can be asymmetric and involve complex morphogenetic phenomena (Figure 242). Sexual reproduction takes place under adverse conditions and produces resistant zygotes, as in Spirogyra, a very widespread Zygnematophyceae (Figure 243).
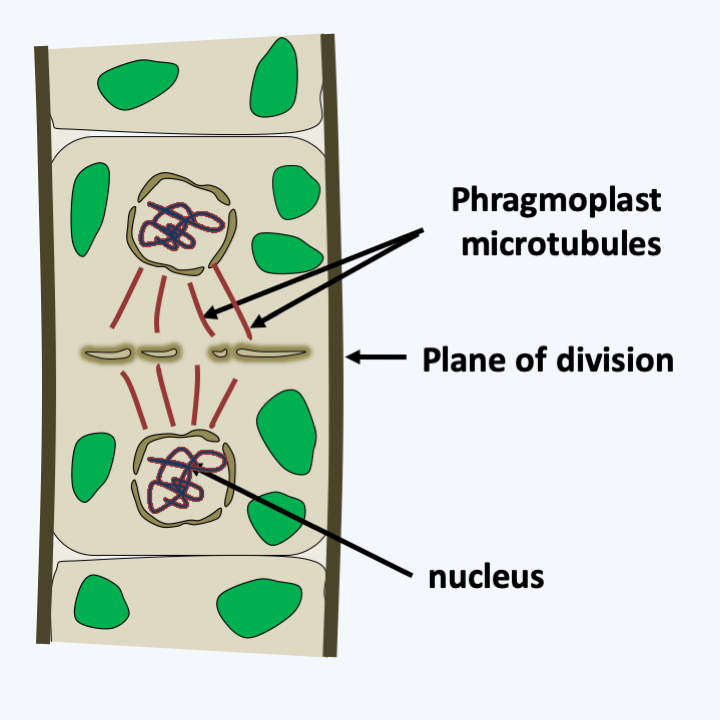
Figure 240.
Division with phragmoplast.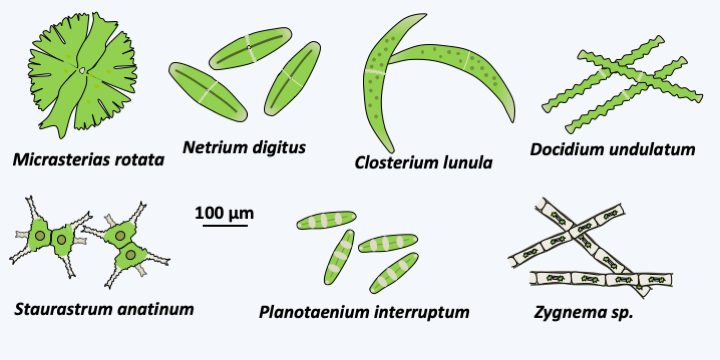
Figure 241.
Diversity of Zygnematophyceae.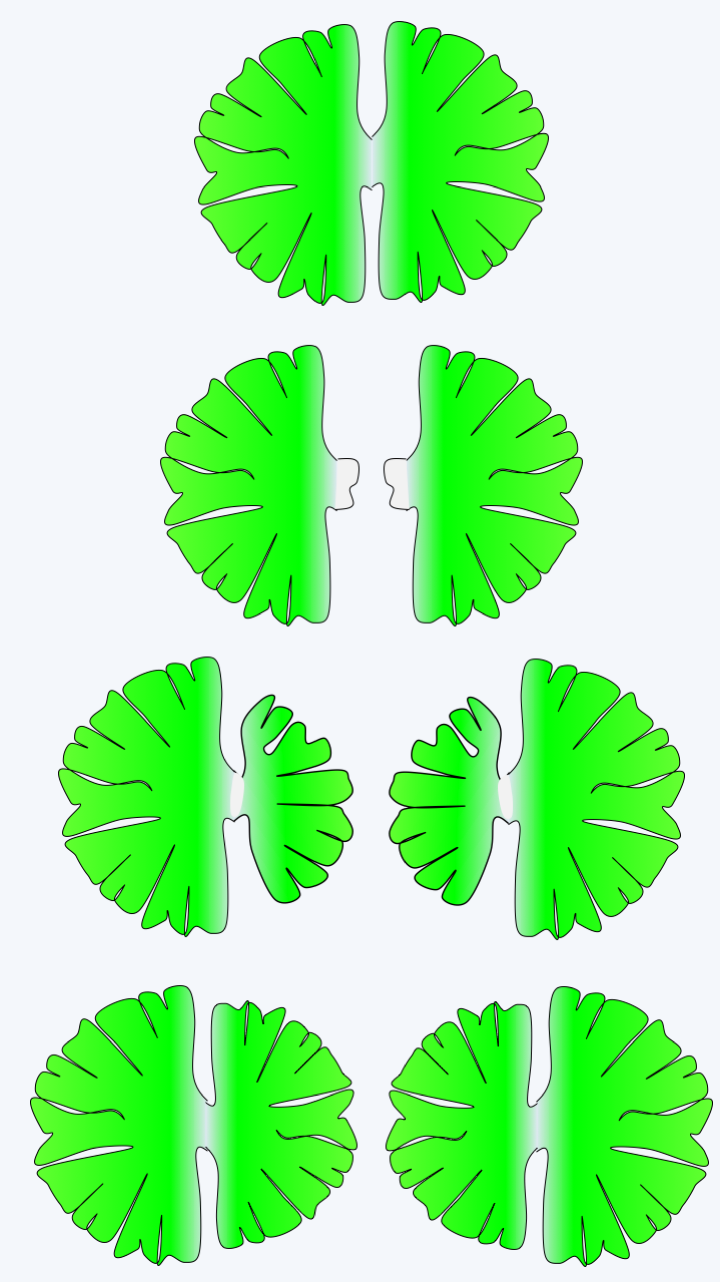
Figure 242.
Division at Micrasterias.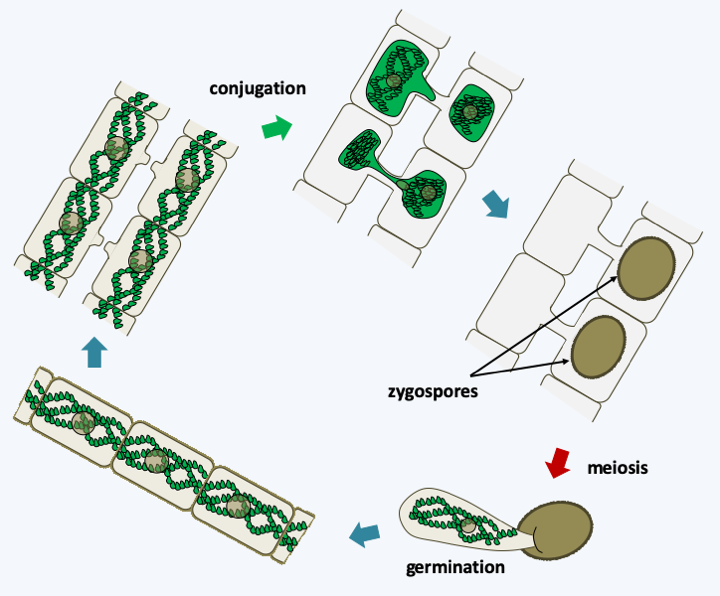
Figure 243.
Spirogyra life cycle. These algae, like other Zygnemarophyceae, have a haplobiontic cycle. Two cells conjugate, their cytoplasm and the nuclei merge to form a zygote which is surrounded by a thick wall. Later, when conditions become favorable again, meiosis occurs and three of the four nuclei degenerate. The zygospore then germinates to give rise to a new haploid thallus. The strains analyzed appear to be homothallic.There are over 700 species of Charophyceae. Their thallus is made up of giant multinucleated cells measuring up to several centimeters in length, arranged side by side to form a tube. New cells form lateral branches which start from nodes arranged regularly (Figure 244). Growth takes place from an apical cell and the algae are anchored in the substrate via a specialized structure formed by translucent rhizoids. Often the thallus is surrounded by a carbonate precipitate of aragonite which gives it a brittle structure. Among the characteristics that bring them closer to land plants, we find the presence of plasmodesmas between cells and many discoid chloroplasts. However, plasmodesmas have a simple structure because they lack the desmotubule, this structure derived from the endoplasmic reticulum and occupies the center of the pore in plants. They therefore resemble the plasmodesmas found in Chlorophyta. We also note the fact that the ovum or oogonium is protected by an envelope of sterile cells before fertilization (Figure 244). Charophyceae have a haplobiontic life cycle similar to that of Zygnematophyceae. From multicellular antheridia (male structures) they differentiate biflagellate sperm. After fertilization, the zygote becomes, like in Zygnematophyceae, resistant structure called an oospore, which allows passing safely through the adverse season. Meiosis takes place just before germination and only one of the four haploid nuclei is capable of dividing into an adult individual, as in Zygnematophyceae. They are also capable of asexual reproduction by differentiating specialized cells from rhizoid cells.
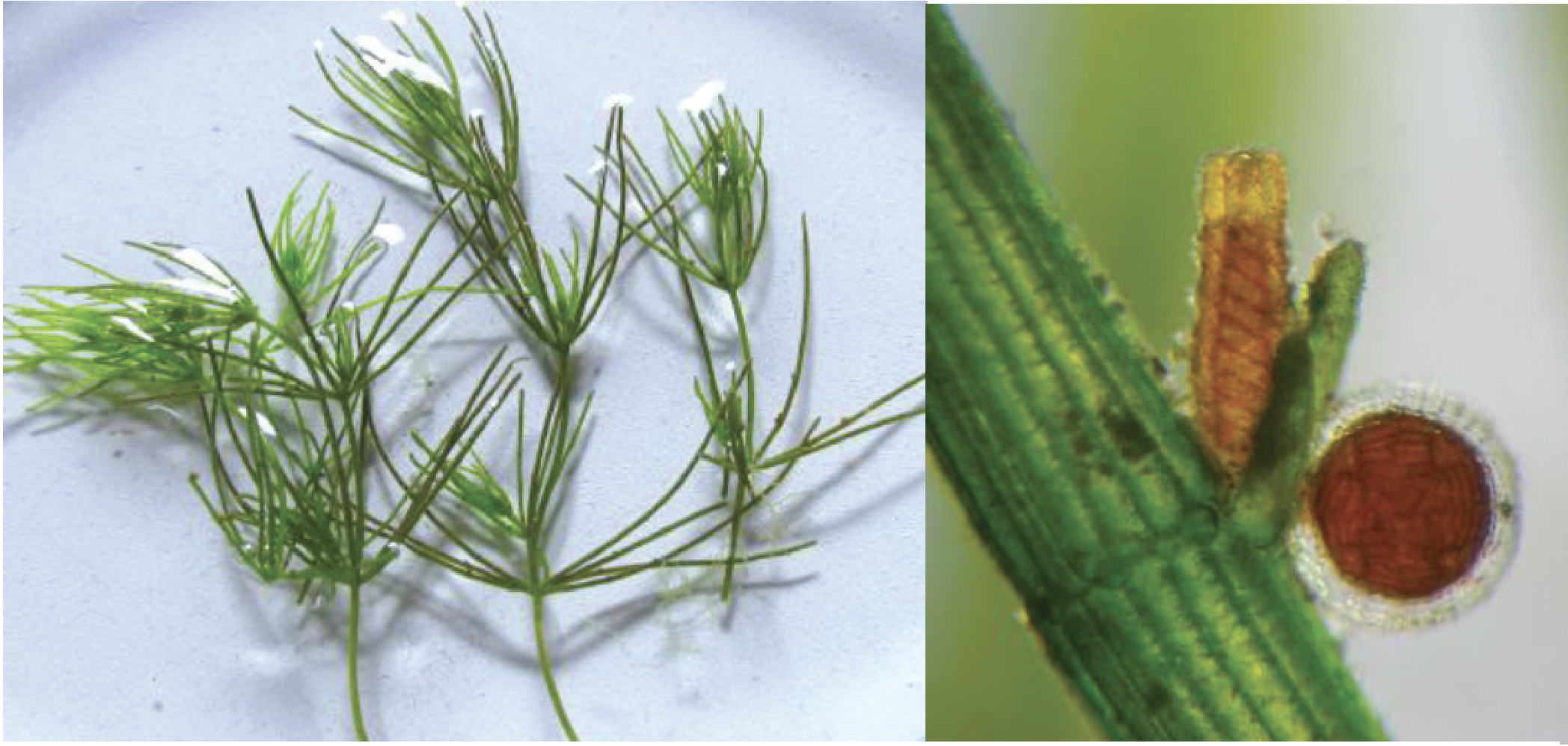
Figure 244.
Thallus of Chara sp. and gamete producing structures. The oogonium is the spindle-shaped structure protected by the spiral whorls. The spherical antheridium located just below contains the biflagellate sperm.Members of the last class of Streptophyta, those of Coleochaetophyceae, have the characteristics most similar to plants. There are about forty species characterized by the presence of filaments, called setae, on their surface, serving as protection against herbivores (Figure 245). Some species are unicellular, the others being either filamentous or composed of a disc with a monolayer of cells. A whole set of biological data indicates that these algae are close cousins of terrestrial plants. There are fossils of primitive plants of the genus Parka which strongly resemble the discoid Coleochaetophyceae. Cells of multicellular Coleochaetophyceae species are connected to each other by plasmodesmas. These plasmodesmas are large pores (20-40 nm) which connect the cells to each other. Extensions of the reticulum, similar to desmotubules, seem to pass through these pores, therefore making it possible to have the lumen of the reticulum in common for two connected cells. These extensions probably serve to transport small molecules and water between cells. Coleochaetophyceae synthesize cell walls comprising cellulose and lignin. They also have the ability to synthesize sporopollenin, cutin and various phenolic compounds found specifically in plants. Other characters, linked to the life cycle, also show a resemblance to plants (Figure 246). In particular, the cells surrounding the zygotes divide to give a layer of protective and nurturing cells. This protective tissue resembles the nourishing tissue of land plants, which have given them the name “embryophytes”.
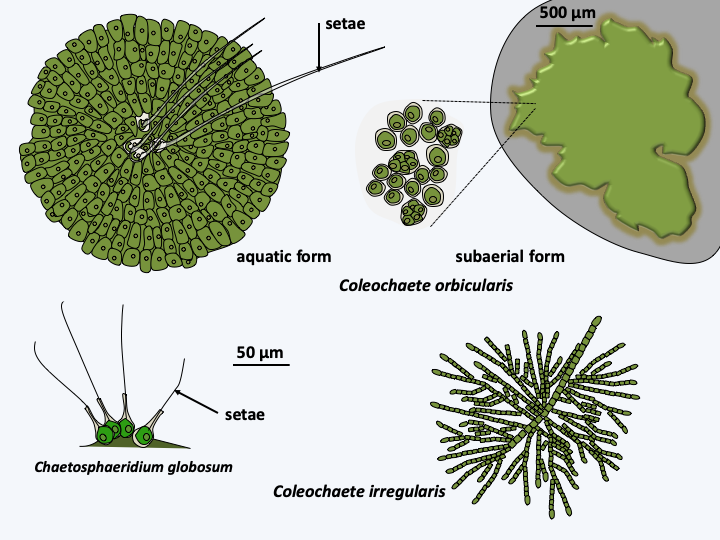
Figure 245.
Some Coleochaetophyceae.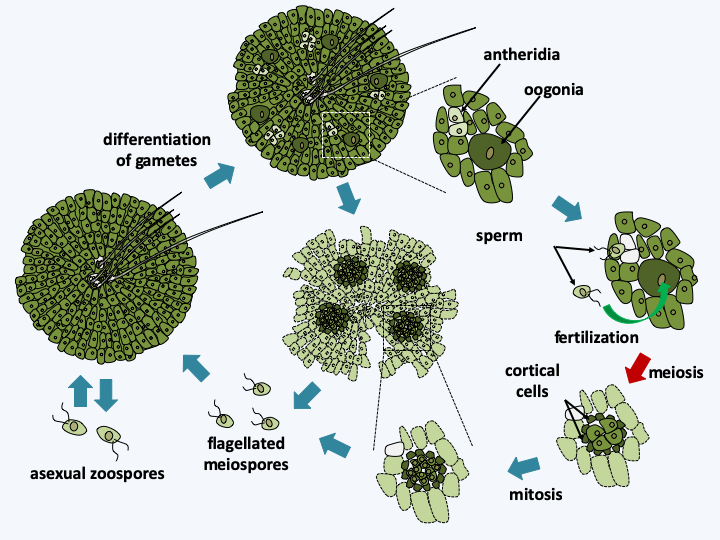
Figure 246.
Coleochaete orbicularis life cycle. The cycle is haplobiontic. Oogonia and biflagellate spermatozoa are differentiated on the haploid thalli. Sperm are produced in antheridia. Only one sperm is produced per antheridium. What makes them unique and close to plants is the fact that after fertilization, the formed zygote remains protected by the parental thallus in chambers bordered by protective and nurturing cortical cells. However, unlike plants and Charophyceae, protection is put in place after fertilization. Then, the zygote undergoes meiosis which is followed by a few postmeiotic mitoses to give rise to several biflagellate spores (between 8 and 32) which will give birth to haploid thalli. These algae are also capable of asexual reproduction through the formation of motile biflagellate cells which can swim and regenerate new thalli.Like Klebsormidiophyceae, Zygnematophyceae, Charophyceae and Coleochaetophyceae are able to resist various stresses including desiccation. Coleochaete orbicularis can differentiate between two types of thalli depending on the conditions, liquid culture or culture in a humid medium such as a humidified quartz surface. The aquatic thallus and the aerial thallus differ in their structure (Figure 245). Cells from aerial thalli retain their properties after a week’s exposure to air and light. When put back into the water, the aerial thalli easily differentiate zoospores. In this species, sporopollenin and lignin allow zygotes and meiospore mother cells to go through poor conditions, including periods of drought. It therefore seems that these algae have already acquired some of the characteristics that would have allowed them to escape from the water. Unfortunately, no genome sequence is available for the three classes of algae closest to plants, preventing a systematic analysis similar to that performed on Klebsormidium flaccidum.
Embryophyta
The Embryophyta, most commonly called “plants”, thus probably derive from algae pre-adapted to aerial life, as they probably lived in fresh water that dried out regularly. Their evolution is closely linked to their exit from water, which probably occurred only once, 450 Ma ago. Indeed, we find the first fossils resembling primitive plants dating from this period, and studies of molecular phylogeny suggest a sole origin for all current plants. Several physicochemical factors in terrestrial ecosystems probably encouraged the emergence of plants from the water. The light required by photosynthesis is more intense, carbon dioxide is more available and mineral salts more abundant above water, even if it was necessary to extract the latter from soils, which was probably not very suitable for the start. It is remarkable to note that the escape of plants from water is concomitant with the establishment of endomycorrhizae with the fungi of the Mucoromycotina and Glomeromycota types. These endomycorrhizae can be found with the majority of plants, including early diverging lineages such as liverworts (see Figure 47). Only a few lineages of plants, like the Brassicaceae, can do without, suggesting a late disassociation between these plants and their endomycorrhizal fungi. It is therefore highly probable that these fungi were important players in plant colonization of land. Some researchers believe fungi to have been decisive in this process. Endomycorrhizae likely improved in the delivery of water from soil, through fungus to plant, probably thus also allowing better absorption of mineral salts.
To get out of the water, however, the plants had to solve several specific problems in the air environment. In addition to resistance to desiccation, probably already present in their algal ancestors, they had to manage gravity, because they are no longer in an environment of the same density, ultraviolet rays, rapid changes in heat and various other turbulences that are more pronounced in air than in water. The absence of water is also particularly crucial for the dissemination of gametes and/or zygotes. To get around these problems, plants have developed biosynthetic pathways involved in cell wall strengthening and/or stress resistance. Some of these pathways seem to pre-exist in charophyte algae, such as the activation of the biosynthetic chains to produce lignin, cutin and various phenolic compounds involved in stress resistance. They were thus covered with an impermeable cuticle secreted by epithelial cells. The pollen and the spores have been covered with sporopollenin which protects them and thus promotes their dispersion in the air. The cell walls were reinforced with lignin, which allowed growth in height towards the light. From a metabolic point of view, their photosynthesis is adapted to withstand strong light through the production of cyclic fluxes of electrons. These are created by two systems, one already present in Klebsormidium flaccidum and resembling NADH dehydrogenase, and the other using the PGR5 protein which also appears to be present in other Chlorophyta, Rhodophyta and Glaucophyta algae.
Plants have many signaling pathways that allow them to respond in a precise way to many stimuli in order to manage the constraints of the air environment. Their ancestors used hormonal systems to regulate cell growth and differentiation, paving the way for complex multicellularity. Additionally, they set up the internalization of gas exchange by developing special structures, the stomata, forming pores to regulate the inflow and outflow of air. Their development culminates with the emergence of vascularization systems facilitating the transport of nutrients and growth in height. The thallus becomes a “corm” made up of roots, stems and leaves, linked by a xylem and phloem vascular system. Once again, plant hormones are already present in Klebsormidiophyceae.
For efficient reproduction in air, the Embryophyta have developed reproductive organs that are protected from desiccation because they are enclosed in structures, similarly as in Coleochaetophyceae. The life cycle in plants sometimes considered “primitive”, such as mosses and liverworts, is haplobiontic and always involves a mobile male gamete, requiring water. It therefore probably resembles that of the ancestors to all land plants. During evolution, there is a progressive modification of the life cycle, tending to increase the diploid phase and the disappearance of the motility of the male gamete. The male gamete is generally dispersed by the wind in the gymnosperms and by insects in the angiosperms.
Analysis of charophyte algae shows that they have many adaptations to the terrestrial environment and that these have simply been “perfected” by plants. It is likely that many lineages of algae living in environments that dried out periodically had properties that allowed them to escape from the water. Why only plants came out with such success is still a mystery. Was a single factor determinant, e.g. the co-evolution with Eumycota fungi, or were multiple factors necessary? The Embryophyta is one of the most successful groups of eukaryotes. Plants have in fact colonized most terrestrial biotopes and some have returned to colonize fresh or salt water. While the majority are photo-autotrophs, many lineages are parasites that no longer photosynthesize. Plants produce a considerable biomass and have diversified in a fantastic way because there are currently around 250,000 species. Their exit from the water had a major impact on the biosphere and particularly on the air environment. It has resulted in increased energy and nutrient flows in terrestrial and freshwater ecosystems, therefore having significant repercussions on these ecosystems, probably participating in the evolution of animals. Likewise, the accumulation of lignin, cellulose and other compounds degradable by fungi has had an impact on their evolution. In the air environment, plant colonization of land was accompanied by atmospheric changes such as the decrease in atmospheric carbon dioxide. It is believed, for example, that the plant roots facilitated acidification and soil disruption, allowing calcium and magnesium silicates to crumble, which could then combine with CO2. The solubilization of silicates would also have favored the evolutionary explosion of diatoms that we have observed in the seas since the beginning of the Tertiary!
Back to chapter index