Multicellularity
Back to main indexIn previous chapters, we have seen that eukaryotes are the result of the assembly of different cells and that these associations between cells of different species continue to occur in the biosphere. Some researchers believe that these associations could also have led to another characteristic of the eukaryotic world: multicellularity. However, the majority of biologists believe that this is not the result of associations between genetically different cells but between genetically identical cells. Multicellularity can be defined as the condition of an organism composed of several differentiated cells for which the activities of the different cells are coordinated. Note that some researchers decouple this definition into (1) multicellularity defining organisms having several cells and (2) multicellularity characterizing organisms having several differentiated cells whose activities are coordinated. In this context, multicellular organisms are not clearly different from colonial organisms in which all the units are identical. We can therefore define unicellular organisms as spending their entire life in the form of a single cell, colonial organisms as those resulting from the association of identical cells and multicellular organisms as those possessing a form made up of several cells, during at least one of the stages of their life cycle.
Multicellularity can be simple, that is to say involve only an often reduced number of cells having elementary intercellular communications and presenting few different forms, most often only two: a “germinal” cell line involved in reproduction and the other, “somatic”, ensuring the functioning of the organism as a whole (Figure 52). Multicellularity can also be complex, and involve sophisticated intercellular communication within tissues containing several cell types, such as in animals, plants or fungi. Note that some complex multicellular organisms, for example humans, also differentiate between germline and somatic cells.
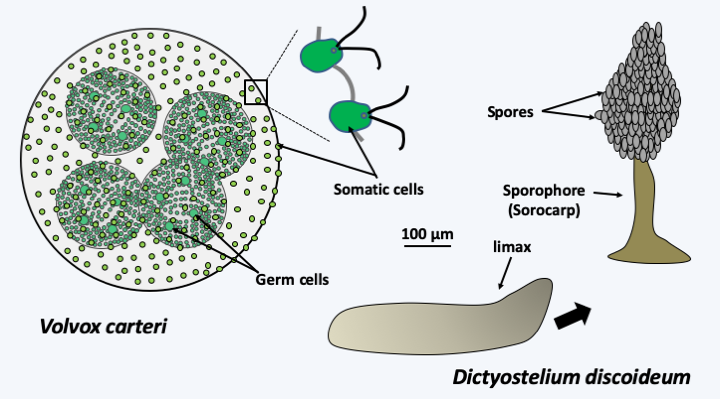
Figure 52.
Two examples of simple multicellularity. Volvox carteri is a freshwater green algae. It is multicellular but differentiates only two cell types: somatic cells resembling Chlamydomonas arranged in the shape of a sphere and larger germ cells which form daughter spheres by mitosis. Amoebozoa Dictyostelium discoideum lives most of its life cycle as isolated amoebae. During asexual reproduction, these cells come together and produce a multicellular organism, the limax or pseudo-plasmodium. This crawls on the substrate and will position itself on the surface of the litter where Dictyostelium discoideum lives. It then turns into a sporophore, often called a sorocarp in such organisms, which looks like a small fungus. The foot of this sporophore consists of apoptotic cells.A study of the size of living organisms based on the study of fossil traces shows that the difference between the smallest and the largest organism is of the order of 1016. The increase in size seems to have occurred in two major stages: (1) the appearance of the eukaryotic cell and (2) appearance of multicellularity of eukaryotes (Figure 53). Recently, large organisms (about ten centimeters;Figure 53andFigure 54) have been discovered that are -2.1 billion years old, therefore probably older than the first eukaryotes, showing that multicellularity appeared early in the course of evolution. However, this exception appears to be unique at this time and the nature of the organisms that created these structures is unknown. It is still possible that they belong to a group that is neither prokaryotic nor eukaryotic today, or that they are primitive eukaryotes.
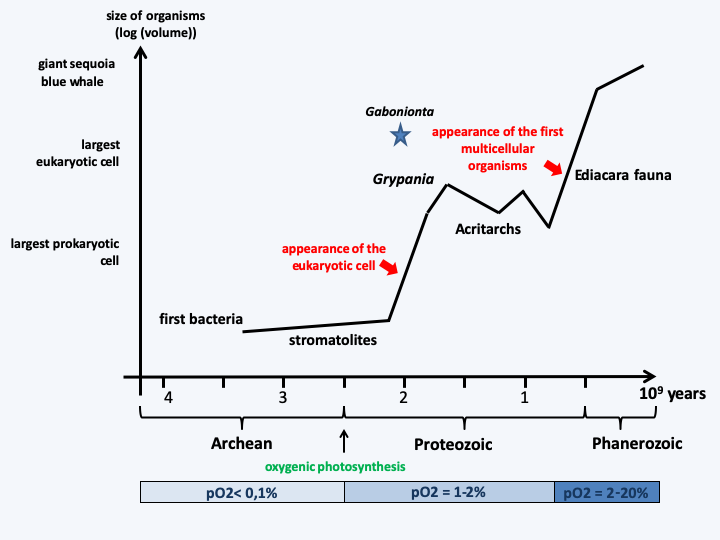
Figure 53.
Evolution of the size of organisms. Stromatolites are colonial cyanobacteria fossils, acritarchs are probably theca fossils protecting eukaryotic cells, and the Edicara fauna are the earliest known animal fossils.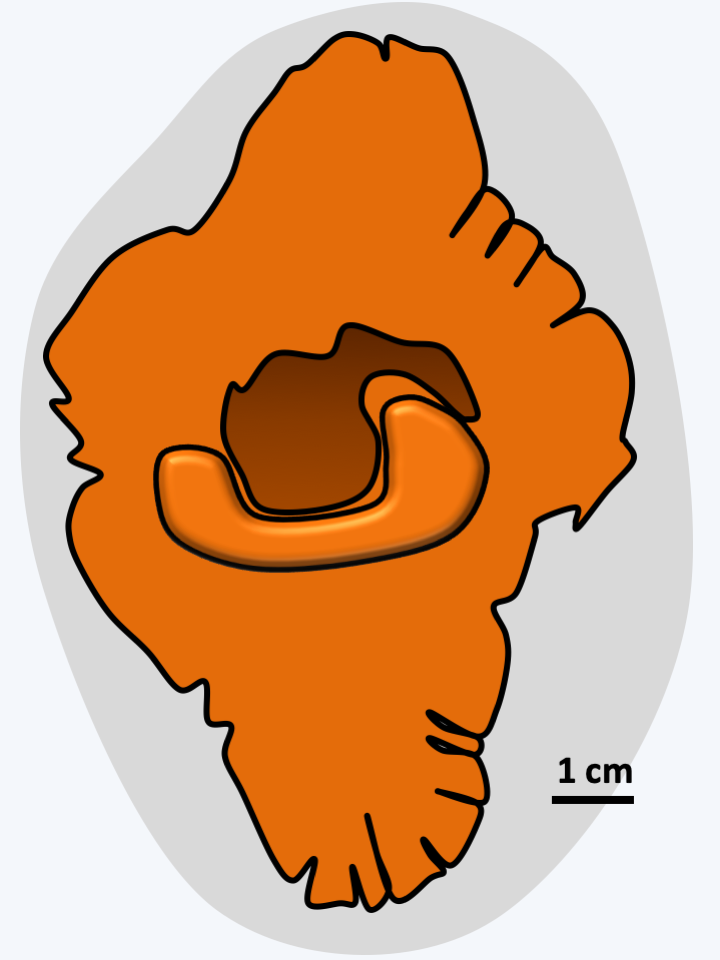
Figure 54.
Fossil group from Franceville or Gabonionta. These large fossils (up to 17 cm) were found in Gabon in a geological layer dating back 2.1 billion years. Their onset follows the increase in oxygen in the atmosphere observed after the occurrence of oxygenic photosynthesis. The presence of complex organisms at such an early date is still poorly explained: complex prokaryotes, the first multicellular eukaryotes or a group that has disappeared without leaving any descendants?Multicellularity is therefore a theme that is essentially specific to the evolution of eukaryotes. Indeed, while there are bacteria capable of forming simple multicellular thalli such as certain cyanobacteria, Myxococcales of the δ-proteobacteria group and streptomycetes, prokaryotes have never produced complex multicellular organisms. However, this is a true multicellularity as there are simple chemical communications within these bacterial thalli to coordinate their development and behavior and in some cases true cellular differentiations are observed. For example, the cyanobacterium Nostoc differentiates a filamentous thallus comprising two types of cells, vegetative cells which provide oxygenic photosynthesis and heterocysts which provide atmospheric nitrogen fixation (Figure 55). The reason for this differentiation is that the enzyme responsible for nitrogen fixation is very sensitive to oxidation. Under no circumstances can this fixation occur in a cell that emits oxygen. The cells appear as chains of photosynthetic cells interrupted by heterocysts whose differentiation involves PatS, a diffusible protein playing a role in communication between cells. The metabolites are exchanged via protein micro-channels. Heterocysts are no longer capable of division; the Nostoc therefore has the beginnings of somatic line/germline differentiation. The chains of Nostoc combine in an extracellular matrix to form thalli that are simple but can reach several centimeters in diameter. However, Nostoc and other prokaryotes never differentiate tissues or organs, although bacterial biofilms look tissue-like in some ways, they do not have the same degree of integration as eukaryotic tissues.
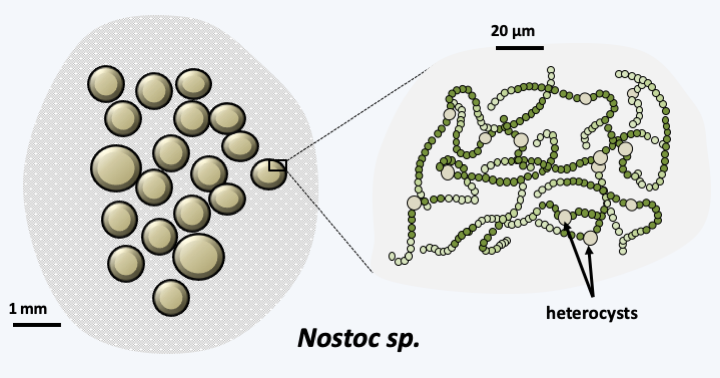
Figure 55.
Nostoc, a multicellular cyanobacterium. Terrestrial cyanobacteria of the genus Nostoc are capable of forming gelatinous colonies of different shapes (spheres, quirky clusters, etc.). Colonies survive desiccation well; they become flat and more or less brittle. After rehydration, the colony regains its shape and metabolism. This is decoupled in two different cell types. Heterocysts fix nitrogen, smaller cells photosynthesize. These cyanobacteria have therefore adopted a simple multicellularityIn eukaryotes, coloniality and multicellularity have appeared several times in phylogenetically very disparate groups and to varying degrees (Figure 56). Some groups are essentially multicellular, such as Oomycota, Phaeophyta, Embryophyta and Metazoa.
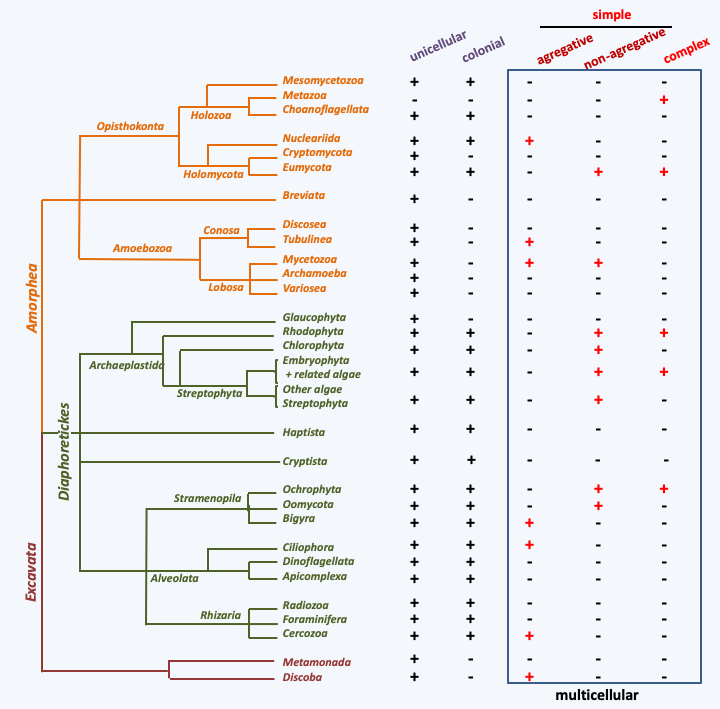
Figure 56.
Evolution of multicellularity in eukaryotes. The presence of the various types of organization in the major eukaryotic lineages is denoted by a +.It is therefore plausible that the common ancestor of each of these groups was multicellular. For other taxa, there are unicellular, colonial and multicellular species. This is the case, for example, with Rhodophyta, Viridiplantae and Eumycota. In these cases, the evolution of multicellularity can be complex with recurring losses and gains. A striking case is that of the Viridiplantae of the order of Chlamydomonadales (Figure 57), in which multicellularity has been invented many times and in which closely related species are multicellular (Volvox and Pleodorina), colonial (Gonium, Pandorina, Volvulina and Eudorina) or unicellular (Chlamydomonas). The phylogeny of these different forms is complex and shows that the unicellular/colonial to multicellular transition has occurred several times (Figure 57). The multiplicity of gain and loss of coloniality and multicellularity in eukaryotes is supported by molecular phylogenies (Figure 56). It is also confirmed that multicellularity evolved in different ways in different lineages, by the fact that in the different colonial/multicellular groups, the extracellular matrix, maintaining the cohesion between the cells, is composed of distinct components: collagens and other proteins in Metazoa, pecto-cellulosic walls in Viridiplantae and chitino-glucanic wall among the Eumycota, etc. These findings suggests that multicellularity arose through different mechanisms and under varying selection pressures. Indeed, the selection pressures brought into play for the establishment of multicellularity are very diverse. Often it is a question either of differentiating cell types which will make it possible to perform a variety of tasks better, or of allowing an increase in the size of the organism, in particular in response to predation (Box 12). A funny example is the one postulated for the Volvox. It appears that these algae, like unicellular Chlamydomonas, are heavier than water and must constantly swim to find themselves in the correct region of the water column necessary for efficient photosynthesis. In Chlamydomonas, cell division, and the associated cytoskeletal rearrangement, stops swimming, and therefore the algae sinks. It can therefore no longer photosynthesize for a short time. It also cannot escape its predators. This is not the case for Volvox because the division of labor between swimming somatic cells and germ cells ensures constant flotation. This mechanism could in fact be very general because many cell types cannot manage both the maintenance of a flagellum and that of a mitotic spindle, both often having the same microtubular origin. The cooperation of a germline ensuring reproduction and a somatic line ensuring movement would then be crucial, to escape predators. However, as Volvox did not replace Chlamydomonas in ponds, interacting selection pressures are not so straightforward (see Box 12). Division of labor and escape from predators are not the only pressures possible. For example, in fungi, the appearance of multicellularity is more likely related to the need to propagate in the terrestrial environment. For these organisms, the spores are more easily dispersed the higher their starting point is. Some fungi differentiate giant cells that can measure more than 10 cm for this purpose. However, these cells are fragile and the wind can lay them down easily. Other fungi are able to differentiate more solid multicellular sporophores which can reach higher into the aerial environment. Finally, it has been shown that in Viridiplantae of the Ulvophyceae family, molecules of bacterial origin participate in morphogenesis. In Choanoflagellata, the formation of colonies (or rosettes) is caused by the secretion of sulfonolipids by bacteria of the phylum Bacteroidetes which are the usual prey of these flagellates. These very surprising observations suggest that the establishment of multicellularity may also be a response to the presence of potentially dangerous microorganisms, or other microorganisms serving as prey. The evolutionary reason for this mechanism is currently mysterious but could be linked to a stress response.
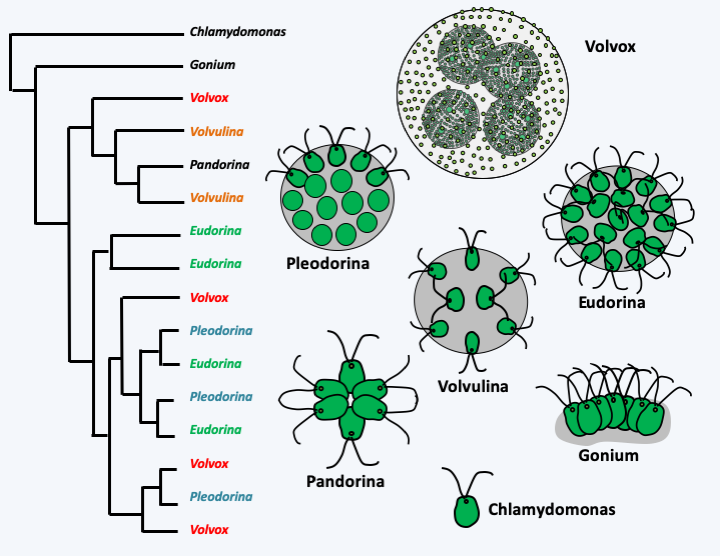
Figure 57.
Phylogenetic relationships in some Chlamydomonadales. The forms Volvox, Pleodorina, Volvulina and Eudorina, formerly considered as genera, have evolved several times in the algae Viridiplantae of the order of Chlamydomonadales, as indicated by the left molecular phylogeny based on the ribosomal RNA sequence.Box 12. Experimental establishment of multicellularity
Two experiments under conditions of strong selection pressure shed some light on the mechanisms capable of generating 'multicellularity'.
In the laboratory, the Viridiplantae alga Chlorella vulgaris reproduces in a strictly unicellular form. The situation changes if it is subjected to predation by Ochromonas vallescia, a Chrysophyta algae. This latter alga is heterotrophic although it possesses a plastid, because the plastid is incapable of ensuring photosynthesis. In a few generations (between 10 and 20), the algal population is dominated by 'multicellular' algae which result from incomplete separation after mitosis between the cells of Chlorella vulgaris. These remain grouped in packs of 8 individuals on average. This change is probably genetic because if the Ochromonas are removed, the algae will remain multicellular for many generations. However, they are quickly replaced by isolated cells under certain lighting conditions, in particular when there is an alternation of days and nights. The interpretation is that predators cannot consume colonies that have become too large, leading to such colonies having a selective advantage. On the other hand, these colonies are at a disadvantage for photosynthesis, probably because the cells shade each other. Note that during the experiment, the authors did not see any modification of the predator that might have adapted to eating larger prey. These results lead to a question: Why are chlorellae unicellular in nature? In fact, all predators not only have a maximum size to capture prey, but also a minimum. For example, Ochromonas valescia rejects prey smaller than 3 μm. It is therefore probable that under natural conditions, colonies of eight chlorellae, but not the isolated chlorellae because they are too small, will fall prey to other predators potentially larger and more voracious than Ochromonas valescia. This experiment therefore indicates that, under the pressure of predation, the establishment of a colonial organism with several cells can be very fast (10-20 generations), but also explains why there are still unicellular beings!
The second experiment consisted of selecting individuals of the yeast Saccharomyces cerevisiae for their ability to quickly sediment, initially by simple gravity and then by centrifuging for 10 seconds at 100 G. Quickly, that is to say after about 60 stages of selection (which can be done in two months if a daily selection is made), spherical colonies in the shape of a snowflake and comprising several dozen cells dominate the cultures. These have defined sizes. Colonies result from the incomplete separation of the daughter cells that have budded, but not from the aggregation of cells via, for example, flocculation proteins. The 'snowflakes' are therefore genetically homogeneous. Colonies reproduce by fragmentation and not by the division of an initial cell. For fragmentation to take place, the colonies must have reached a critical size, which shows that the colony has a juvenile stage, during which it cannot reproduce, and an adult reproductive stage. Finally, after about thirty selection steps, cellular genotypes triggering apoptosis in certain cells allow better regulation of the size of the colonies, giving them optimal sedimentation/fragmentation rates. In fact, the larger the clusters, the faster they sediment, but the less they reproduce. The apoptotic cells being more fragile, they will facilitate the genesis of daughter colonies. So a division of labor quickly developed with certain cells that 'sacrifice' themselves in order for the colony/organism to reproduce more efficiently.
Two main types of mechanisms have been put forward to explain the appearance of organisms made up of several cells, whether they are colonial or truly multicellular. First, there are organisms that pass the vegetative period of their life cycle as independent cells. After a stimulus, these cells will come together to form an organism, sometimes possessing several million cells and which in some species can appear in the form of a slug endowed with motility, the pseudo-plasmodium or limax (Figure 58). Once positioned correctly, this set of cells metamorphoses into a sporophore, the sorocarp. This coloniality/multicellularity is said to be aggregative. Its complexity appears to be maximal in Dictyostelida of the phylum Amoebozoa. In fact, in Dictystelium discoideum (Figure 52) and related species, the pseudo-plasmodium endowed with motility consists of at least three differentiated cell types, with cells called to become spores called pre-spore cells, cells which will apoptose to give the stem of the sorocarp, the pre-stem cells of which there are three subtypes, and immune cells responsible for eliminating the potentially pathogenic bacteria present in the pseudo-plasmodium called S cells. During morphogenesis, pre-spore cells organize as a polarized epithelium. Despite these characteristics, the aggregative multicellularity that has appeared at least seven times during evolution (Figure 56) remains simple and has not produced complex multicellular organisms. This was produced only by the other type, the non-aggregative multicellularity. In this type, cells arise from the division of a cell whose daughters remain attached to each other (Figure 59). This is typically what happens in organisms with a complex multicellularity that results from the development of a zygote or, as in fungi, the proliferation of cells. The initial cell may divide in the classic way or else undergo schizogony (Figure 59). In the first case, as in aggregative multicellularity, coloniality/multicellularity results from the adhesion of cells to each other, while in the second case, it results from the fragmentation of a plurinuclear organism, an event often referred to as cellularization, because it gives rise to classical cells with a fixed number of nuclei (often one). These two mechanisms are widespread in the living world. For example, embryonic development in vertebrate eggs follows the first mode, while that of insects in its early stages follows the second mode. Schizogony is present in many protists (Myxogastrida, Foraminifera, Plasmodium…) which are not all multicellular. Indeed, this division can occur after a long vegetative phase like that of a plasmodium actively seeking its prey (Figure 59). These giant cells can reach twenty centimeters in diameter and contain billions of nuclei. In (Myxogastrida, they transform into a multicellular sporophore, while in Foraminifera they cellularize in the form of independent cells. Myxogastrida are therefore considered to be multicellular organisms while Foraminifera are not. In Pezizomycotina fungi where the zygote remains plurinucleated, a similar cellularization mechanism is in play, which produces cells with two nuclei which will continue a development involving particular divisions and which will culminate in the formation of asci. These are included in a multicellular fruiting body whose cells are of maternal origin.
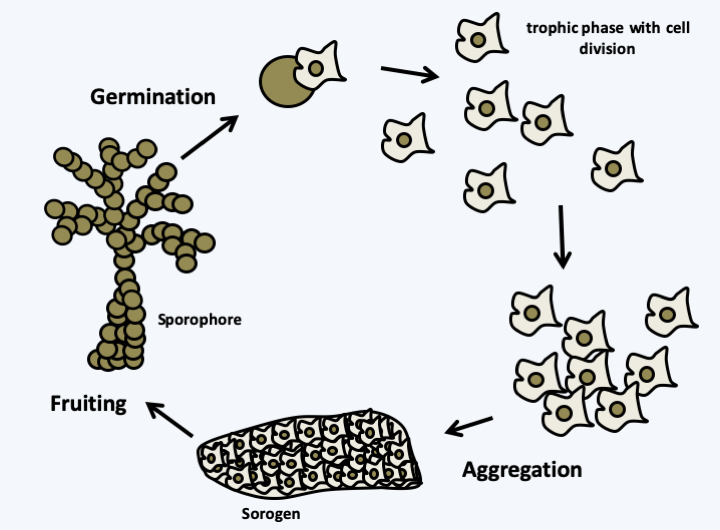
Figure 58.
Aggregative multicellularity. Organisms with aggregative multicellularity spend part of their life in unicellular form. This is the time when they take in food and the cells divide. A stimulus, often nutritional deficiency, will trigger their aggregation in the form of a self-sustaining multicellular sorogen. The sorogen continues its development by transforming itself into a sporophore which will carry spores. The size and shape of the sporophores vary widely, but are usually less than 1 mm. When placed in the right conditions, the spores germinate and give rise to isolated cells, marking a return to the unicellular state. In this type of multicellularity, genetically different cells can assemble, which can give rise to conflicts where certain genotypes seek to maximize their chance of dispersal for the benefit of others, for example by devoting fewer cells to make the foot of the sporophore.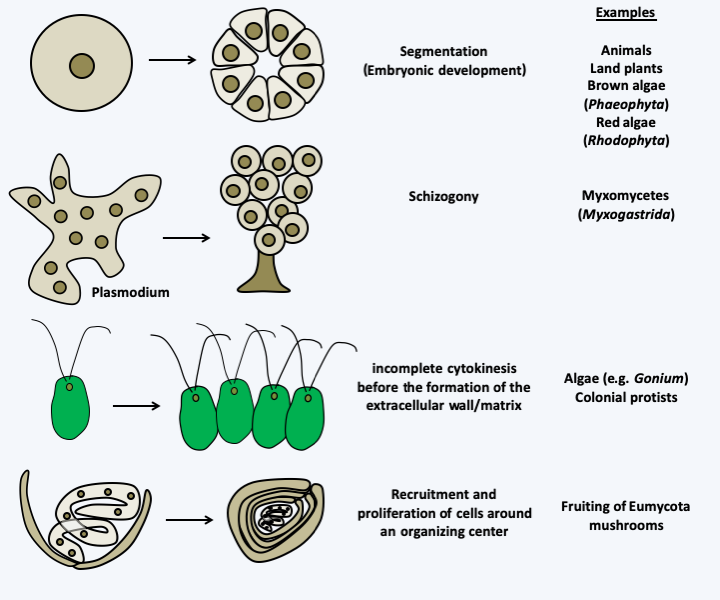
Figure 59.
Non-aggregative multicellularity. This type of multicellularity can result from several different mechanisms, the main types of which are represented. In all cases, it results from the cohesion of cells resulting from the same ancestral cell (or in some cases, from several cells as for the development of fruiting in fungi). If all cells are derived from a single mother cell, they are genetically homogeneous (there are few cases described where differentiation is accompanied by genetic modifications), which eliminates conflicts between cells.Once made up of multiple cells, colonial organisms only become truly multicellular if those cells differentiate from each other to perform different tasks. These differentiated states are often associated with more or less long-distance communications that reinforce the integration of different cells within the multicellular organism. For each group of multicellular organisms, the molecules involved and the networks in which they are inserted are different. However, there are molecules that seem to be reused repeatedly: homeodomain transcription factors, MAP kinase pathways, modified histones, small RNAs, hormones, protein growth factors, etc. Differentiations are at their peak in animals, but they are present in many organisms, including organisms that are considered to be predominantly unicellular, such as yeast. However, the term differentiation hides many meanings. For example, in a colony of yeasts or bacteria, such as those seen on Petri dishes, there are differentiated states that form through gradients of nutrients, oxygen, or waste such as ammonium. Likewise, several protists have the ability to change shape depending on environmental conditions, for example switching between an amoeba form in dry environments and a flagellate form when water is abundant. If these states cannot be considered as true terminal differentiations, that is to say irreversible and definitive for a cell which will no longer change, such as those present in organisms with complex multicellularity, they call upon the same molecular mechanisms for to set up. Indeed, with rare exceptions, differentiated states do not result from genetic changes but from changes in gene expression. Counter-examples are, among others, the ability of different lymphocytes in mammals to secrete a specific type of antibody which involves deletions of part of the immunoglobulin locus or the passage between the MAT a and MAT α mating types in Saccharomyces cerevisiae which involves a gene conversion event. The changes in expression can be reversible and rather fall within the scope of regulatory events, or difficult to reverse, or even irreversible, and can be considered as true terminal differentiations.Figure 60shows how particular interactions in regulatory networks can lead to reversible regulatory phenomena, irreversible differentiated states or even oscillating phenomena as in rhythmic phenomena. The differentiated states are characterized by the presence of autocatalytic regulatory loops or positive feedbacks leading to the accumulation of certain molecules. The irreversibility is more or less strong depending on the modifications made to the regulation networks, often in relation to the number of positive feedback loops. For example, in animals, the dedifferentiation of cells is impossible without making genetic modifications; in plants it is often necessary to remove the wall to return to the totipotent state; in fungi, all cells are often considered totipotent and without terminal differentiation because they can regenerate the entire body simply if they are isolated from others and re-inoculated on a nutrient medium. Nevertheless, there seem to exist irreversibly differentiated cells incapable of dividing within the fruiting bodies of fungi, e.g. in the cell lineage leading to asci. The differentiated states are therefore mostly caused by epigenetic and non-genetic modifications. In protists, if some of these modifications occur at the chromatin level as in animals and plants, there are examples in different species based on modifications of signaling networks (“epigenetic switches” in yeasts based on alternative states of expression of transcription factors, antigenic variability linked to the sequestration in particular regions of the nucleus of genes encoding surface proteins, sectors and related phenomena in fungi, at least one of which is linked to irreversible activations of MAP kinase pathways, etc.) or changes in the structure of proteins such as prions in yeasts and filamentous fungi, or molecular scaffolding, among others in paramecia (see Box 21). In summary, eukaryotic protists set up mechanisms as sophisticated and based on the same molecular mechanisms as “higher eukaryotes” to differentiate cells, whether unicellular or multicellular.
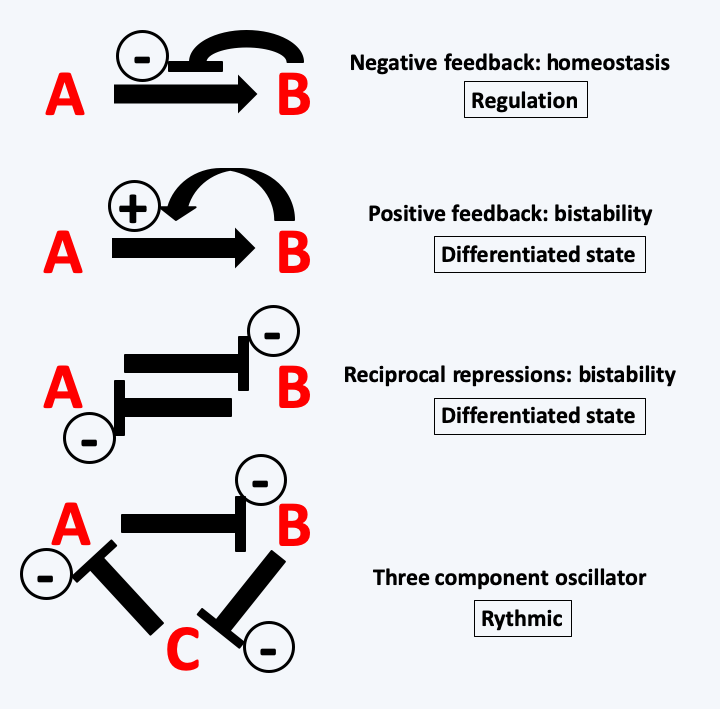
Figure 60.
Possible interactions in biosynthetic or regulatory networks. Molecules within cells interact with each other and can generate so-called 'emerging' phenomena, that is, having special properties additional to the simple addition of interacting molecules. For example, when the product B of a reaction inhibits its own synthesis by interacting with the enzyme catalyzing the reaction from A to B, this leads to homeostasis of the amount of that molecule even if the concentration of substrate A fluctuates. This mechanism is frequently encountered in cells because it is an important mechanism in the regulation of cell homeostasis. On the contrary, when product B promotes the catalysis of its production, this leads to differentiation. In general, catalysis only occurs when substrate A reaches a critical threshold. The cell cannot therefore contain too much A, otherwise the reaction starts and B appears. The same type of phenomenon occurs if A and B mutually inhibit their own synthesis: a cell will not be able to contain both A and B. The fact that it instead contains A or rather B is based on the initial concentrations of A and B and the intensities of the repressions. Cell differentiation is based on the accumulation of such feedback / repression loops. Their numbers determine the stability / reversibility of the differentiations. More complex relationships between molecules lead to other emerging phenomena that are often difficult to predict. For example, three molecules which sequentially repress their synthesis will cause an oscillatory behavior of their production and therefore to the creation of rhythms.InFigure 53, it can be seen that the two jumps in size of organisms during the history of the biosphere seem to be correlated with an increase in the oxygen level in the atmosphere. While the appearance of the eukaryotic cell via mitochondrial endosymbiosis seems to be a response to survive the presence of O2, the appearance of complex multicellular organisms, such as animals, seems to have occurred only when the pressure in oxygen was sufficient. Indeed, it is probable that in the first multicellular organisms there was no system for distributing oxygen, like our blood system. Only a pressure greater than 2% could allow the cells located in the center of large clusters to breathe properly. This may be one of the reasons why despite the diversity of the mechanisms that led to coloniality and then to multicellularity, there is an enzyme that seems to be a marker of it. It is not absolute because there are several exceptions, but in general, multicellular organisms have NADPH oxidases, while unicellular ones do not. These enzymes are membrane-bound and generate extracellular superoxide ions from molecular oxygen by consuming NADPH (Figure 61). They are therefore ideal for setting up intercellular signaling which connects the metabolic state of a cell via the concentration of NADPH to a diffusible short-lived signal affecting neighboring cells: extracellular superoxide ions. Some researchers believe that these enzymes are also able to detect oxygen pressure. This could allow cells to locate their position in a multicellular cluster. The innermost cells being the least in contact with oxygen and therefore producing the fewest superoxides. This will allow a harmonious differentiation of structures and tissues. It therefore seems that despite a recurrent emergence of multicellularity, it could have initially used the same signal: the oxygen pressure coupled with the capacity to produce biomass because the NADPH used mainly in anabolic reactions measures anabolic activity. This hypothesis seems to be confirmed in fungi because these enzymes are often absent in species living only as yeast, while being necessary for the development of fruiting bodies in multicellular Ascomycota and Basidiomycota. More recently, it has been shown that the inactivation of the genes encoding these proteins in Dictyostelium discoideum leads to defects in the multicellular stage of this organism with aggregative multicellularity, whereas inactivation in the unicellular amoebae have no defect, further confirmation of this hypothesis. Once this first type of communication was in place, more efficient signals based on small molecules (hormones) or proteins (hormones and growth factors) took over.
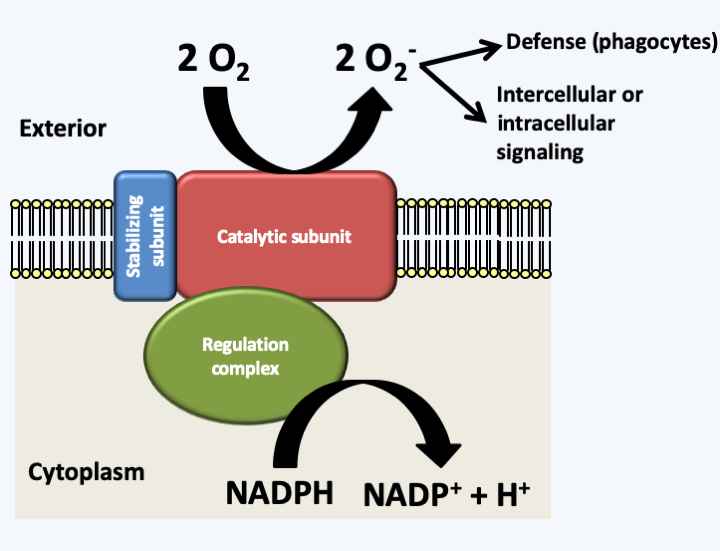
Figure 61.
Structure and function of typical NADPH oxidase. NADPH oxidases are plasma membrane enzymes capable of producing superoxide ions using electrons from NADPH. Superoxide ions are produced outside the cell or in vesicles whose interior is the topological equivalent of the exterior of the cell. These ions can either be used to destroy other cells (this is the reaction phagocytes initiate to destroy foreign bodies or plant cells during the hypersensitive response) or be used for signaling. There are isoforms, especially in plants, where the regulatory complex and the stabilizing subunit are absent and are replaced by calcium response sequences located at the N-terminus of the catalytic subunit.The next step in the complexification of eukaryotic organisms appears to be the association within a society of individuals, much like in social animals. In protists, these societies, although less complex, seem to exist. Seen from a certain angle, organisms with aggregative multicellularity are societies of cells. As in all societies, there are cheaters in these organisms who will try to maximize their reproduction to the detriment of other cells. The analysis of the behavior of these cells could reveal variants of these behaviors, possibly applicable to more complex societies such as animal or human societies. Likewise, in 2011, primitive agriculture was described in Dictyostelium discoideum. Until this discovery, agriculture and animal husbandry were the prerogative of human and animal societies, as with ants for example. In Dictyostelium discoideum, some strains seem not to eat all the bacteria available, which are then incorporated into the sporophores. The bacteria are dispersed with the spores and then serve as an inoculum to initiate new “cultures” of bacteria suitable for feeding the amoeba. This behavior is not present in all strains. There is therefore a dichotomy, like what happens in human societies, between hunter-gatherers and farmers!
In conclusion, the repeated emergence of multicellularity where identical cells associate, like symbiosis where different cells unite, is a major theme in the evolution of eukaryotes (Figure 62). Its acquisition was a prerequisite for the evolution of animals and plants. If there are a few cases where it seems to have regressed, as in certain yeasts for example, on the whole, evolution seems to have rather led to its repetitive appearance and its improvement, which suggests that the more complex multicellular organisms are in many biotopes better equipped to win the race for evolution than their unicellular cousins. All the events of symbiosis and the acquisition of multicellularity have led to a very complex evolution of eukaryotic cells, of which we do not yet know all the details.Figure 62is only a very simplified representation of major events!
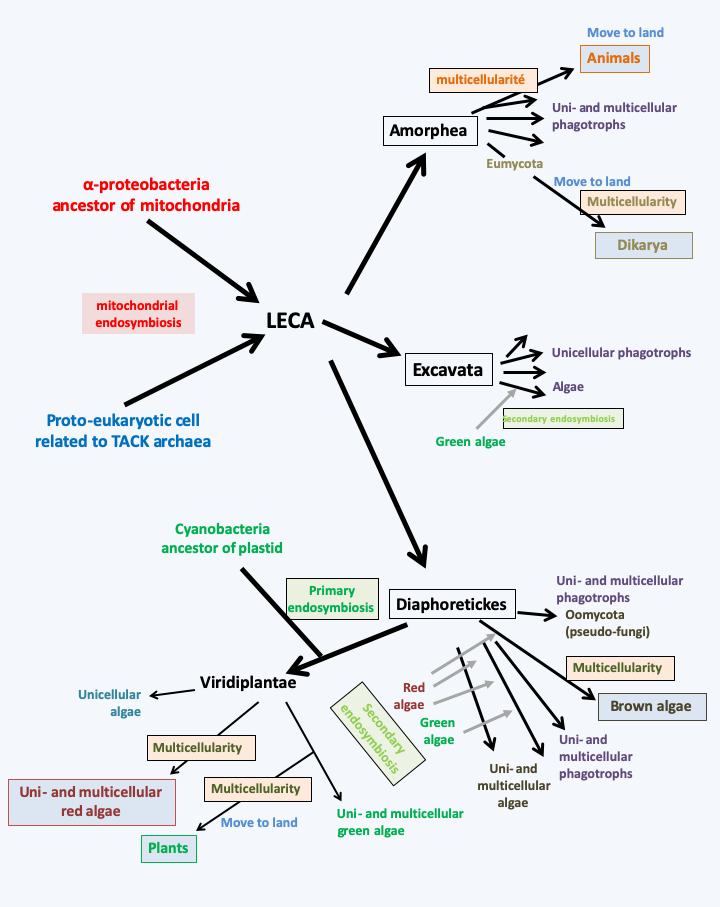
Figure 62.
Summary of important stages in the evolution of eukaryotes. Only major endosymbiosis events leading to complex multicellularity are represented. The story begins with mitochondrial endosymbiosis, probably two billion years ago. The phylogenetic analyses, explained in the second part of the book, indicate that three major lineages quickly diverged after this event that created eukaryotes. That of Excavata underwent a relatively simple evolution, apparently marked by a single secondary endosymbiosis event involving a Viridiplantae. No organism in this group appears to have acquired multicellularity. That of the Amorphea did not experience endosymbiosis leading to the establishment of plastids, but was marked by the recurrent acquisition of aggregative multicellularity. In this group, the complex multicellularity evolved once in the ancestors of animals and probably twice in dikaryotic fungi. The last line called Diaphoretickes went through a very complex evolution. Primary endosymbiosis and multiple secondary endosymbiosis have generated large numbers of algal lineages from phagotrophs, resulting in a mosaic of related groups feeding either by phagotrophy, photosynthesis, or both. One group, the pseudo-fungi, even suffered loss of phagotrophy and the acquisition of the fungi's particular mode of nutrition (osmotrophy). Aggregative or non-aggregative multicellularity has appeared over and over again in various lineages of Diaphoretickes. The complex multicellularity has evolved at least three times: in red algae (Rhodophyta), in green algae (Viridiplantae) and in brown algae (Phaeophyta).Back to chapter index